Graphical abstract

Highlights
- •
Direct laser metal sintering (DLMS) 3D printing conditions were optimized for medical-grade Ti-6Al-4V powders.
- •
The inner defect amount and mechanical properties varied depending on the laser spacing (30–100 μm).
- •
An optimally fabricated 3D-printed titanium alloy had similar biological capacities to machine-cut counterparts for dental applications.
- •
The potential usefulness of 3D printing technology was suggested for multiple dental devices.
Abstract
Objective
Titanium and its alloys are widely used for dental and medical biomaterials due to their excellent mechanical and biological advantages. After the introduction of direct laser metal sintering (DLMS) 3D printing technology and its use over conventional machine-cut processes, questions remain regarding whether 3D-printed titanium (alloy) devices have similar biological properties to machine-cut counterparts for dental applications. Thus, this work focuses on comparing the biological activities of machine-cut and 3D-printed specimens after optimizing the DLMS 3D-printing conditions in terms of the mechanophysical characteristics.
Methods
The DLMS 3D-printing (as a function of the laser spacing from 30–100 μm) and post-surface treatment (as-given or sand-blasted) conditions were optimized using medical-grade Ti-6Al-4V powders in terms of the inner pore amount, mechanical properties, roughness and hydrophilicity. Then, the initial cell adhesion of the optimized DLMS 3D-printed Ti-6Al-4V specimen was compared with that of the machine-cut Ti-6Al-4V specimen against human dermal fibroblasts (hDFs) and mesenchymal stem cells (hMSCs), which are representative of direct-contact cell types of orofacial mucosa and bone, respectively. hMSC differentiation on the specimens was conducted for up to 21 days to measure the osteogenic gene expression and biomineralization.
Results
Laser spacings of 30–40 μm had fewer inner defects and consequently a higher three-point flexural strength and elastic modulus compared to other larger laser spacings. Depending on the span width (0.3–1 mm) in the lattice architecture, the elastic modulus of the 3D-printed cuboid specimen can be further controlled (up to ∼30 times). The sand-blasted specimens after 3D printing revealed lower surface roughness and higher hydrophilicity compared to the as-3D printed specimen, which were considered optimal conditions for biological study. Initial hDF and hMSC adhesion for 12 hr and hMSC differentiation on the surface were comparable between the sand-blasted 3D-printed and machine-cut specimens in terms of adherent cell numbers, vinculin intensity, osteogenic gene expression and biomineralization.
Significance
The optimized DLMS 3D-printed Ti-6Al-4V specimen had similar biological properties to those of the machine-cut counterpart, suggesting the potential usefulness of 3D printing technology for a wide range of dental applications.
1
Introduction
Biomaterials used in dental and medical areas must demonstrate appropraite mechanophysical and biological properties to achieve a high degree of biofunctionality . Titanium and its alloys are widely used for dental and orthopedic applications due to their low density, excellent mechanical properties and great biocompatibility . Until now, titanium and its alloys have generally been produced for medical use by machining titanium rods or plates by computer numerical control (CNC) machines and subjecting them to various surface modifications to improve the mechanical properties and biological response . Thus, machine-cut titanium and its alloys with appropriate surface modifications have become widely-used biomaterials in clinical settings .
Within the last 15 years, direct laser metal sintering (DLMS) as a 3D printing technology was introduced as a promising fabrication technology for titanium and its alloys . Then, it became commercially available due to its great reproducibility, good customizing ability for obtaining complex architectures with controllable surface geometry, cost effectiveness for the fabrication of individual parts and relatively good resolution (∼20 μm), which attracted a myriad of dental applications, such as implantable devices (i.e. dental implants and scaffolds) and prosthetic devices (i.e. dental crowns and denture frameworks) .
The main merits of utilizing DLMS 3D-printed titanium (alloy)-based medical devices are the excellent mechanical properties due to the low density and versatility of the architectures obtainable to mimic the natural anatomical 3D geometries of hard tissues . For even accelerating osteogenesis, the micro or macro porosity, surface roughness, and drug loading capacity can be further optimized to accelerate biological response, while maintaining the mechanical properties . However, questions remain regarding whether optimized 3D-printed medical devices have similar biological capacities to machine-cut counterparts, especially for dental applications .
Therefore, the aim of this study is to compare the biological activities of machine-cut and 3D-printed specimens after optimizing the 3D-printing conditions. To achieve this target, DLMS 3D-printing (as a function of the laser spacing from 30 to 100 μm) and post-surface treatment (as-given or sand-blasted) conditions were optimized using medical-grade Ti-6Al-4V powders (grade 5) in terms of the mechanophysical properties, such as the inner defect levels, mechanical properties, roughness and hydrophilicity. Then, the biological effects of optimized DLMS 3D-printed Ti-6Al-4V specimens were compared with those of machine-cut Ti-6Al-4V specimens against human dermal fibroblasts (hDFs) and mesenchymal stem cells (hMSCs), which are representative direct-contact cell types of orofacial mucosa and bone, respectively. Thus, the first null hypothesis of this study is that there are no differences in the mechanical properties relative to the laser spacing (30–100 μm). The second null hypothesis is that there are no differences in the biological activities, such as the cell adhesion and osteogenic capacity of stem cells, between the optimized 3D-printed and machine-cut specimens.
2
Materials and methods
2.1
Specimen preparation
All specimens used in this study except for the as-provided machined-cut specimens used for the biological assay control group were prepared by selective laser melting 3D printing technology. The particle size of the Ti-6Al-4V powder (grade5, Advanced Powders & Coatings Inc. QC, Canada) was measured by a particle size analyser (LA-950V2, HORIBA, Kyoto, Japan), and the images and compositions of the powder were obtained by field emission scanning electron microscopy (FE-SEM, Sigma 300, ZEISS, Oberkochen, Germany) on an instrument equipped with energy-dispersive spectroscopy (EDS) (Oxford). The specimens were fabricated in an argon atmosphere, in accordance with the output conditions (radiation power of 225 W and scanning rate of 1200 mm/s) using a DLMS 3D printer (SM250, Sentrol Co., Ltd, Seoul, Korea) with a Yb (Ytterbium) fiber laser wavelength of 1060 nm. To optimize the 3D printing conditions, the disk specimens (10 mm diameter and 2 mm thickness) were fabricated according to the different laser spacings (30, 40, 50, 60, 70, 80, 90, or 100 μm). The minimum value for the laser gap was set at 30 μm based on average diameter of Ti6Al4 V powder (∼37.5 μm) where the laser gap for DLMS should be similar or larger than the powder diameter to sinter two individual powders together for 3D-printing. All of the specimens, except the lattice structure, were 3D-printed with a 45-degree axis. To test the mechanical properties, bar specimens (25.0 × 4.0 × 2.0 mm) and lattice structures (10.0 × 10.0 × 20.0 mm) with different span widths (0.3, 0.5, 1 mm or block type) were prepared. The single lattice sizes were 3, 4, and 5 mm for the 0.3, 0.5, and 1 mm span width groups, respectively. The bar specimens were made from different laser spacings (30, 50, 70, and 90 μm) for the three-point flexural strength test, and the lattice structures were made with a 50 μm laser spacing. The edges of the bar specimens were chamfered with P600 SiC paper and sand-blasted with 50 μm alumina at 4.3 bar pressure using a sandblaster (Basic Classic, Renfert, Hilzingen, Germany) for 60 s to clean the whole surfaces. Lattice structures with different gaps were made for varying the elastic modulus of the Ti-6Al-4V construction. For the surface characterization and cell experiments, disk specimens (with a 10 mm diameter and 2 mm thickness) were made with a 50 μm laser spacing. The disk specimens were divided into 3 subgroups: ‘as 3D-printed’, ‘3D + polished’, and ‘3D + sand-blasted’. The as 3D-printed group was not surface-treated after 3D printing. The 3D + sand-blasted group was sand-blasted on one surface for 60 seconds with 50 μm alumina at 4.3 bar pressure using the aforementioned sandblaster. The 3D + polished group was polished with P220-SiC paper to obtain a flat surface. The completed specimens were serially cleaned for 5 min each in acetone, ethanol and distilled water in an ultrasonic chamber ( Table 1 ).
Method | Manufacturing process | Experimental parameter | Detail conditions | Specimen types |
---|---|---|---|---|
Fe-SEM analysis for pore detection | 3D-printing | Laser gap | 30, 40, 50, 60, 70, 80, 90, or 100 μm | Disk (d = 10 mm and h = 2 mm) |
Flexural strength and fractography | 3D-printing | Laser gap | 30, 50, 70, or 90 μm | Bar specimens (25.0 x 4.0 x 2.0 mm) |
Modulus from lattice architecture | 3D-printing (50 μm laser gap) | Lattice span width | 0.3, 0.5, or 1 mm | Lattice structures (10.0 x 10.0 x 20.0 mm) |
Surface analysis | 3D-printing (50 μm laser gap) | Post-surface treatment after 3D-printing | As printed, sand-blasting, or polishing | Disk (d = 10 mm and h = 2 mm) |
In vitro analysis | 3D-printing (50 μm laser gap) or machined-cut | Manufacturing method | 3D-printing or machined-cut | Disk (d = 10 mm and h = 2 mm) |
2.2
Surface pores and total pore area in cross-sectioned specimen
The disk specimens (with a 10 mm diameter and 2 mm thickness) with different laser spacings (30, 40, 50, 60, 70, 80, 90, and 100 μm) were vertically embedded in epoxy resin (EpoFix Kit, Struthers, Ballerup, Denmark) for visualizing the cross-sectional plane to detect surface pores and total pore area. The specimens were serially polished using a polisher (LaboPol-5, Strouers, Ballerup, Denmark) with P220 ∼ 4000-grit SiC papers. After the specimens were cleaned with distilled water and coated with Pt, scanning electron microscopy (SEM, JSM-6510, JEOL Ltd., Tokyo, Japan) images were captured. The surface pore numbers and total pore area were measured by using an image measurement program (ImageJ version 1.46, USA) at 3 sites per specimen and then averaged ( n = 3) . The minimum value of the pore area was set at 0.4 mm 2 .
2.3
Mechanical properties and fractography
The flexural strength of the bar specimens (25.0 × 4.0 × 2.0 mm) with different laser spacings (30, 50, 70, and 90 μm) was measured by the three-point flexural strength test at a 20 mm span length ( n = 5) using a universal testing machine (Instron 5966, Instron, MA, USA) at a crosshead speed of 1 mm/min. The three-point flexural strength ( σ , N/mm −2 ), was calculated by the following formula ( σ = 3 PL /2 wt 2 ), where P is the maximum flexure load (N), L is the length of the support span (20 mm), w is the specimen width (mm) and t is the specimen thickness (mm). Fractography analysis of the broken bar specimen was performed to investigate the fracture behaviors using SEM or FE-SEM to track the possible starting point during fracture. The elastic modulus of the lattice structure (10.0 × 10.0 × 20.0 mm) was measured by compressive strength analysis using Bluehill software (version 3.31, Instron) according to the span width (0.3, 0.5, 1.0 mm or dense block type).
2.4
Surface characteristics of the specimens for the in vitro study
The surface of the disk specimens for the in vitro study was observed using SEM for the different surface treatments (as 3D-printed, 3D + sand-blasted, 3D + polished). The surface roughness was measured using a surface roughness analyser (SJ-400, Mitutoyo, Kawasaki, and Japan) to measure Ra (arithmetical mean), Ry (maximum height), and Rz (height irregularities, n = 5). Scan was conducted at a speed of 5 mm/s with measurement width (4 mm) and cut-off values (0.8 mm). The average value of three measurements is reported as the representative value for each specimen. The hydrophilicity of the specimen surfaces was measured by contact angle analysis using a Surface Electro Optics instrument (PHX300, SEO, Suwon, Korea) and distilled water (10 μL and n = 5).
2.5
Cell culture
hMSCs (PCS-500-012, ATCC, Manassas, VA, USA) were cultured in a bone marrow-mesenchymal stem cell growth medium (ATCC) that was supplemented with 7% fetal bovine serum (FBS, Gibco, Grand Island, NY, USA), 15 ng/ml recombinant human insulin-like growth factor (rh IGF-1), 125 pg/ml recombinant human fibroblast growth factor (rh FGF-b), 2.4 mM l -alanyl- l -glutamine and 1% penicillin/streptomycin (Gibco) according to the recommendations provided by the manufacturer. Human dermal fibroblasts (hDF, PH10605AK, Genlantis, San Diego. CA, USA) were cultured with high glucose Dulbecco’s modified Eagle’s medium (DMEM) supplemented with 10% FBS and 1% penicillin/streptomycin. The cells were cultured in a humidified atmosphere of 5% CO 2 at 37 °C .
2.6
Initial cell adhesion test
To investigate the initial cell adhesion ability on different Ti-6Al-4V specimens (machine-cut, as-given specimen after CNC cutting without 3D printing technology, or 3D + sand-blasted specimen), 200 μL of the hMSC or hDF cell (2 × 10 4 ) suspensions was placed on top of the specimen with the above specific growth media. 3D + sand-blasted specimen was chosen after consideration of hydrophilicity and 3D printing needs for maintaining complex architectures, which cannot be attained via other post-treatment conditions. Machine-cut without any surface treatment (grade5, Kobelco, Kobe, Hyogo, Japan) and 3D + sand-blasted specimen from powder (grade5, Advanced Powders & Coatings Inc. QC, Canada) are all from Ti-6Al-4V of grade5 with a 10 mm diameter and 2 mm thickness. After 4 h, 800 μL of the growth media was added, and incubated for 8 h. Then, the cells were fixed with ice-cold 4% paraformaldehyde for 30 min. The fixation solution was then removed, and all specimens were washed with phosphate-buffered saline (PBS). Then, cell membrane permeabilization was conducted for 5 min using 0.2% Triton X-100 (T8787, Sigma Aldrich, St. louis, MO, USA). After being washed with PBS 3 times, all specimens were immersed in 1% bovine serum albumin (BSA) solution (BAH68, Equitech-bio, Kerrville, TX, USA) to block nonspecific protein interactions. The blocked specimens were incubated with an anti-vinculin (1:200, mouse, sc-73614, Santa Cruz Biotechnology Inc, Dallas, TX, USA) primary antibody overnight at 4 °C with 1% BSA. All specimens were washed three times with PBS and then further incubated with rhodamine (TRITC)-conjugated donkey-anti-mouse (1:400, 715-025-150, West Grove, PA, USA) for 1 hr with 1% BSA. Then, 488 phalloidin (1:400, A12379, Thermo Fisher Scientific, Waltham, MA, USA) and 4′,6-diamidino-2-phenylindole (DAPI) (1 μg/ml, 62248, Thermo Fisher Scientific) stains were applied. After the specimens were transferred to a microscope cover glass, vinculin focal adhesion (red), actin (green), and DAPI (blue) were captured with an inverted microscope (Olympus, IX71, Tokyo, Japan) with a 20x objective (Fluor 0.45). In particular, vinculin was observed from five different samples to quantify the fluorescence intensity ( n = 5, ImageJ version v1.52a).
2.7
Stem cell differentiation
The hMSCs (2 × 10 4 ) seeded on the disk specimens (machine-cut, as-given specimen after CNC cutting without 3D printing technology, or 3D + sand-blasted specimens) or 24-well tissue culture plates (TCPs) for 24 h were differentiated with osteogenic media supplemented with ascorbic acid (50 μg/mL), b-glycerophosphate (10 mM), and dexamethasone (100 nM) with a media change every 2–3 days until 14 or 21 days were reached. To investigate the osteogenic capacity of the 3D-printed specimens, real-time polymerase chain reaction (PCR) and Alizarin red S (ARS) staining were performed according to the methodology in the literature . Briefly, at day 14, 1 μg of extracted ribonucleic acid (RNA) was transcribed to complementary deoxyribonucleic acid (cDNA), and quantitative PCR experiments were performed using a real-time PCR machine (StepOne-Plus, Applied Biosystems). The gene expression of osteocalcin (OCN) and osteopontin (OPN) were normalized to that of B-actin. The growth media culture on TCP for 14 days was used as a negative control. The primer sequences used for the real-time PCR analysis were as follows: B-actin, forward 5′-CATGGATGATGATATCGCCGCG-3′ reverse 5′-ACATGATCTGGGTCATCTTCTCG-3′; OCN, forward 5′-GTGCAGCCTTTGTGTCCAAGCAGGA-3′ reverse 5′-CCGTAGAAGCGCCGATAGGCC-3′; and OPN, forward 5′-CCCATCTCAGAAGCAGAATCTC-3′ reverse 5′-ATGGCTTTCGTTGGACTTACT-3′. On day 21, the cells were stained with a 2% ARS solution (pH 4.2) for 30 min , and images were captured by optical microscopy (Olympus lX71, Shinjuku, Tokyo, Japan). All experiments were independently performed in triplicate, and the representative data set and images are shown.
3
Results
3.1
Powder characterization and preparation of specimens using a 3D printer
The Ti-6Al-4V powder size (powder analyser), morphology (FE-SEM) and composition (EDS) were initially investigated ( Fig. 1 A) . The mean powder size and standard deviation were 37.7 ± 15.0 μm. The FE-SEM images revealed spherical particles with a size that was in agreement with the results of the mean powder size. The EDS analysis displayed a typical composition of Ti-6Al-4V with 89.6% Ti, 6.4% Al and 4.0% V (wt%). Various shapes of 3D-printed specimens were successfully produced ( Fig. 1 B).

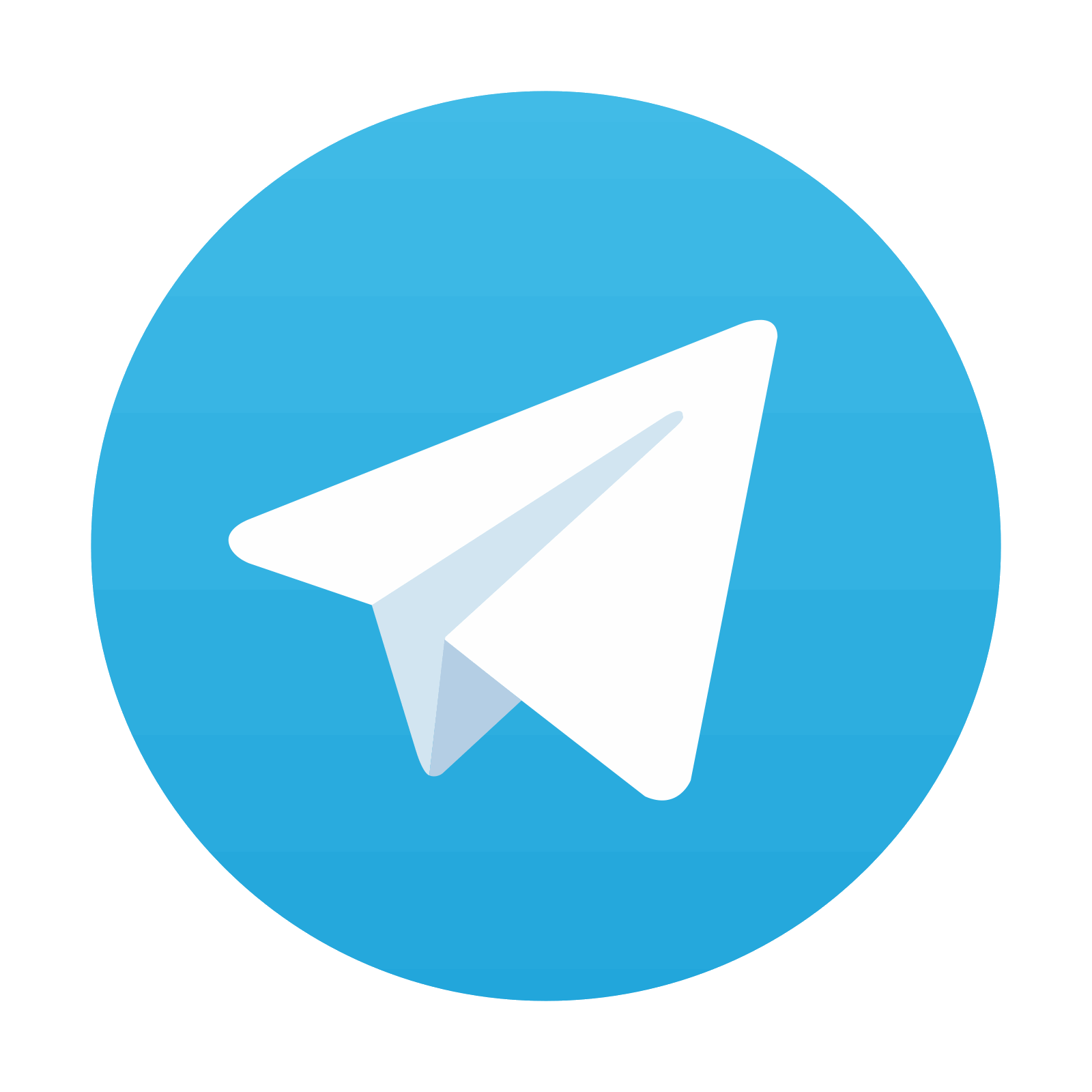
Stay updated, free dental videos. Join our Telegram channel

VIDEdental - Online dental courses
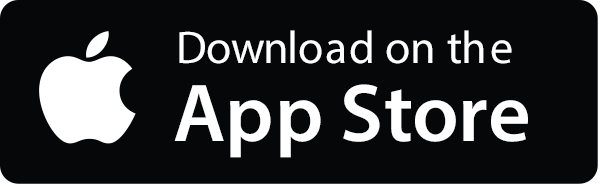
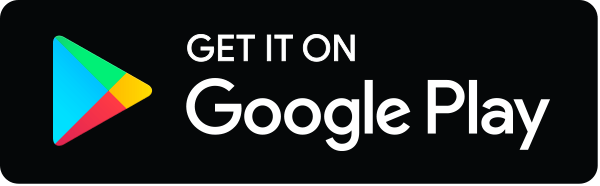
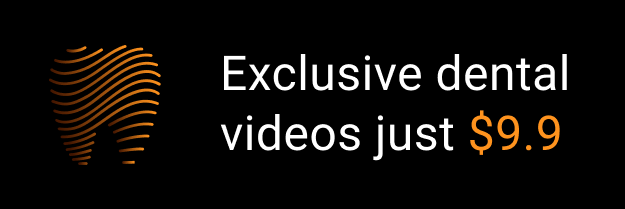