5
Dental Materials in the Digital Age
Geoffrey A. Thompson and Hongseok An
5.1 Introduction
Almost 50 years ago, computer‐aided design–computer‐aided manufacturing (CAD‐CAM) technologies and workflows were introduced to dentistry. Today, there are many CAD‐CAM systems and workflows available for the manufacture of highly accurate dental prostheses and at a relatively low cost. Several systems and workflows allow for design and restoration in the dental office, while others rely on the dental laboratory or a manufacturing center. The benefits of a digital workflow or CAD‐CAM processes include an increase in quality and reproducibility, efficiency, and access to newer and nearly defect‐free materials. The materials used in CAD‐CAM processes can be ceramics, polymers, or metals.
5.2 Materials for CAD‐CAM Prosthodontics
5.2.1 Ceramics
A ceramic is a combination of a metal and a non‐metal. Metals used in the manufacture of dental ceramics include aluminum, sodium, zinc, zirconium, and titanium. Non‐metals include nitrogen, oxygen, and carbon. Ceramics are hard, stable, and strong, but are brittle under tension. Brittleness is overcome by supporting the ceramic with a high‐strength core material or by selecting for a ceramic with increased toughness or flexural strength.
Dental ceramics may be crystalline or non‐crystalline materials or a mixture. There are three main classes of dental ceramics based on their microstructure: (i) glassy; (ii) partially crystalline glass ceramics; or (iii) polycrystalline ceramics (Giordano 1996). Ceramics with a predominantly glassy microstructure, such as leucite and feldspathic based glasses, are more esthetic, but possess the lowest mechanical properties, and are usually employed as veneering materials. While ceramics with a more crystalline phase, such as zirconia and alumina, are stronger, but they are not as esthetic. The strength and esthetic properties of the partially crystalline glass ceramics, such as lithium disilicate, lie between glassy and polycrystalline ceramics.
Ceramic materials contain strength‐limiting flaws, and their actual strength is usually less than the theoretical strength by two orders of magnitude (Holloway 1973). Flaws are classified as intrinsic or extrinsic. Intrinsic flaws are due to grain size mismatch, large grains, phase boundaries, phase transformations, or microcracking from anisotropic contraction or expansion of different crystalline phases (Fairhurst et al. 1994, Rice and Pohanka 1979). Extrinsic flaws are from manufacturing processes and can be voids, machining damage, aggregates, or inclusions. Toward producing high‐strength ceramics, it is important to understand, then control the inclusion of strength‐limiting flaws during processing, and during the manufacture of dental restorations from these materials.
5.2.2 Common Processing Methods
Machinable and pressable CAD‐CAM materials developed for ceramic restoration workflows may result in an increase in quality and reliability of the finished restorations. The starting materials are industrially manufactured and are almost defect‐free before use. However, each fabrication method and laboratory technique produces unique flaw populations with respect to type, geometry, and distribution (Ramadhan et al. 2018). For example, the formation of porosity in different pressable ceramic materials is commonly observed, while abrasive grinding of dental ceramics has been shown to produce cracking and surface defects (Denry 2013, Quinn et al. 2005, Chang et al. 2011) that reduce restoration strength (Hung et al. 2008).
Fully sintered ceramic materials are very hard, and, as a result, they are difficult to mill. There is also an investment in terms of the time required to machine a restoration and for the replacement of worn milling tools. Cracks and chippings are commonly observed in milled fully sintered ceramics and may result in a poorer quality final restoration. It is more common that ceramics are machined in a partially sintered or pre‐crystalline state known as the “green state.” Following milling, the green state restoration receives a crystallization or sintering heat treatment to complete sintering and improve the mechanical properties of the restoration.
5.2.3 Polymers
Polymers are lightweight, ductile, and have a lower strength compared with most metals and ceramics. However, their esthetics and ease of repair make them a useful material for many dental applications. Oftentimes, the high functional loads observed in the oral environment require that classical polymers, used in fixed prosthodontic situations, be reinforced with or supported by a stronger material.
Newer, high‐performance polymeric materials have been introduced for complete mouth reconstructions to include poly ether ether ketone, poly‐aryl‐ether ketone, poly ether aryl ketone, and poly ether ketone. Some of these materials are manufactured with carbon reinforcement or glass fibers. The high‐performance materials are usually used as a supporting framework and require veneering material(s) to mask its color.
Resin composites and high‐density polymethacrylate materials have been developed and may be used for both provisional and definitive restorations. These materials are less brittle and rigid, but possess superior flexibility, fracture toughness, and machinability compared with dental ceramics.
5.2.4 Common Processing Methods
Prior to the introduction of CAD‐CAM workflows, polymers were cast, extruded, or molded. However, innovations have made it possible to use newly developed restorative materials by employing additive and subtractive technologies. These newer technologies enhance the capability of manufacturing prostheses with complex shapes.
5.2.5 Metal Alloys
Metals used in dentistry are almost always an alloy or a combination of two or more metals resulting in a material with improved properties. Typical properties of metals include high strength, polishability, ductility, and opacity. The combination of strength and ductility means that metals can bend many times without fracturing; however, the only metal that possesses an endurance limit or fatigue resistance is steel. Noble and base metals used in dentistry are all susceptible to fatigue; therefore, careful planning of prostheses should account for support and magnitude of expected loads. Dentistry’s greatest challenge when using metals involves hiding them from view by layering with polymers or ceramics.
5.2.6 Common Processing Methods
Historically, restorative metals were cast, forged, or sintered; however, today, most CAD‐CAM workflows are either additive or subtractive. Subtractive manufacturing machines away bulk material in a controlled process until the desired final shape is attained. Some of the advantages of subtractive milling include the capability to mill from a large selection of materials and the surface finish is under the machine operator’s control (Han et al. 2018, Takaichi et al. 2013). Dental restorations can be milled from a spectrum of metal alloys, to include high‐noble, noble, and base metals. Cobalt–chromium and titanium alloys are some of the commonly used base metal alloys (Han et al. 2018, Takaichi et al. 2013). The selection for a machinable dental alloy is based, in part, on material cost and mechanical properties (Han et al. 2018, Baumann et al. 2010). A disadvantage of subtractive milling technology is the millings are usually discarded as waste and may increase production costs (Noorani 2006). The scientific literature does not support CAD‐CAM milling technology over classical laboratory procedures for fabricating a metal restoration and both workflows can result in clinically acceptable restorations (Papadiochou and Pissiotis 2018, McLean and von Fraunhofer 1971). A comparatively newer technology called additive manufacturing (AM) was developed in response to a need for rapid manufacturing or prototyping. Innovations in AM CAD‐CAM workflows have allowed for the fabrication of complex shapes using selective laser melting and electron beam melting. The leading advantage of AM is that waste may be reduced in comparison with subtractive manufacturing methods. In addition, AM is not limited by milling tools or the number of milling machine axes; therefore, very complex dental restorations may be manufactured. The biggest disadvantage of AM may be the cost of initial equipment acquisition.
5.2.7 Reasons for Selection
Some of the reasons for considering a material or combinations of materials for use in a prosthodontic reconstruction may be esthetics, anticipated forces, mechanical properties, available space, wear resistance, and survival rate. Proper testing and evaluation of materials and workflows should always precede performance claims.
5.2.8 Esthetics
Translucency is a function of a material’s transmission, absorption, and reflectance (Figure 5.1). Both metal and polycrystalline ceramic core materials in a porcelain‐veneered restoration can affect the esthetic presentation of a restoration. Core materials affect restoration translucency, especially in the gingival third. Polycrystalline ceramic materials, such as alumina and the most common form of zirconia, do not transmit light and are directly related to the size and frequency of light‐scattering sites, such as grain size, grain boundary area, impurities, inclusions, second phases, and porosity. Glass ceramics and 4 and 5 mol% yttria partially stabilized zirconia fall between the glassy and polycrystalline materials and readily transmit visible light.
5.2.9 Anticipated Stress or Forces
The magnitude of forces on teeth and dental restorations is individual and a function of a patient’s personal habits, both while awake and during sleep. It is also influenced by the direction of force, prosthesis design, and the type of restoration.
Classical polymeric dental materials, such as acrylics and urethanes were unable to withstand high functional forces without support from a high‐strength framework. Sometimes occlusal forces and personal habits can still exceed the strength of the polymers, even when supported by metal reinforcement (Figure 5.2). However, newer high‐performance polymers are produced using reliable industrial production methods, oftentimes, utilizing high heat and pressure in the process. These materials have good flexure strength and can be used to produce complex shapes in a milling machine.

Figure 5.1 Incident light may be transmitted, absorbed, or reflected.

Figure 5.2 Even with metal reinforcement, acrylic demonstrates cracking under functional loads.
Metals possess many excellent properties, including high strength, and are often used in restorative dentistry. Classification of casting metals is based, in part, on their indications for use, that is, inlay/onlay (low stress), short span fixed partial denture (high stress), or a long span fixed partial denture (very high stress). The greater the expected stress, the greater should be the yield strength of the casting alloy. While a spectrum of metals exists for printing and milling dental restorations, cost and material properties strongly influence their selection. Precious metal‐based dental alloys may be printed or milled; however, it is more common to select for a base metal alloy, such as cobalt–chromium or titanium or its alloy. Different CAM processes and digital workflows have been shown to affect mechanical properties, internal fit, and marginal adaptation (Prudente et al. 2018, Önöral 2021, Hong et al. 2022, Ng et al. 2014, Al Maaz et al. 2019, Sulaiman 2020). Other research has demonstrated acceptable or an expectation of acceptable clinical performance of CAM metals (Arroyo‐Cruz et al. 2021, Levartovsky et al. 2021).
There are many dental ceramics available on the market to select from. Expected stress, as influenced by location in the mouth, and prosthesis design will affect selection criteria. One of the determinants for clinical longevity of dental restorative materials is flexural strength. That said, one of the weakest glassy materials, feldspathic porcelain, has a long and successful track record for use in restorative dentistry principally because these ceramics are typically supported by a high‐strength core material or take advantage of adhesive bonding technology. Both procedures, can increase the load necessary to initiate failure without changing the strength of the weaker glass material. In an effort to reduce costs, improve reliability, and increase precision, all‐ceramic restorations are rapidly replacing those containing metal.
Glass ceramics possess remarkable esthetics and may be milled or pressed. They have moderate strength and are usually employed for single‐tooth restorations or short, fixed dental prostheses. Intraorally, load‐to‐failure is increased by dental bonding technology (Malament and Socransky 2010). The polycrystalline ceramics alumina and zirconia are less esthetic but possess sufficient strength to be used as a monolithic structure. Esthetics is obtained with polycrystalline materials by the application of superficial or intrinsic colorants. Alternatively, polycrystalline materials may be layered or veneered with a more esthetic glass or glass ceramic. Polycrystalline ceramics possess the highest flexure strength among the available ceramic materials, and short‐term clinical evaluations suggest that when minimum recommendations are adhered to, clinical success can be expected (Vizcaya 2018).
5.2.10 Mechanical Properties
Two useful terms for understanding the mechanics of dental materials are stress (σ) and strain (ε). Stress is defined as the force per unit area and strain is defined as the deformation in a material when subjected to stress. Strain may be elastic (reversible) or plastic (permanent).
Stress–strain curves show the response of a material to stress (usually tensile stress), and important information for a specific material can be determined (Figure 5.3). The slope of the linear region of the stress–strain curve is the elastic modulus (or Young’s modulus of elasticity) and is a measure of the stiffness of a material. Yield strength is defined as the stress at which a material exhibits a specific amount of plastic strain. The ultimate tensile strength is the greatest stress observed and may be greater than the stress recorded at the point of fracture. The toughness is the ability of a material to absorb elastic energy and to deform plastically before fracturing (measured as the total area under the stress–strain curve).
In dentistry, forces are applied to teeth and to dental restorations through the muscles of mastication. The forces are different depending upon age, sex, personality, habits, condition of the dentition, and the number of remaining dental units. A restorative dentist’s objective should be to reduce the likelihood of strain occurring because of applied stress through design, dimension, angulation, and type of restoration. Offset loads or cantilever loads may be the most detrimental type of force to dental prostheses, whether it is a single or multi‐unit dental restoration. Offset loading produces complex forces and its magnitude is affected by the restorative volume, or the height and width of the restoration, and the length of the offset load. Forces produce micro‐rotations and stress concentrations, and low forces over a long time may result in fatigue failure (Figures 5.4a,b and 5.5a,b). These factors must be considered when selecting materials for use as a prosthetic restoration.
Rigid materials absorb less energy compared with non‐rigid materials and more stress is transferred through the material. Acrylic resins and composites delay force transmission and reduce force peaks and can act as shock absorbers (Menini et al. 2013).

Figure 5.3 Stress–strain curve.
Many studies refute the presence of, or requirement for shock absorption; however, those studies used mechanical tests or finite‐element modeling and do not necessarily simulate mandibular movement and occlusal forces. The periodontal ligament apparatus provides feedback and helps control mandibular movement. This is important because the force transmission to peri‐implant bone is 3–9 × greater. When the damage threshold to bone is not exceeded, bone can remodel, but when the threshold is exceeded, bone resorption may occur (Tokmakidis et al. 2009, Abichandani et al. 2013).

Figure 5.4 (a) A 20‐year‐old gold alloy bar was removed from Branemark external hexagon fixtures; (b) the bar exhibited fatigue cracks through the gold cylinder abutments.

Figure 5.5 (a) Less than two‐year‐old locator fixture; (b) fixture demonstrates physical signs of fatigue before failure.
5.2.11 Available Space
Prosthetic and laboratory manufacturing guidelines suggest minimum dimensions when planning restorations with metal, metal‐reinforced, or all‐ceramic restorations on natural teeth or implants. The 3D space available must be sufficient and may depend upon choice of restorative material. For example, brittle restorative materials such as ceramics, and less‐durable materials like polymers will have different 3D space requirements. When restorative space is insufficient and in a non‐esthetic zone, choosing a metal or a metal‐reinforced restoration can be recommended.
5.2.12 Wear Resistance
The influencing factors on abrasivity are relative hardness of opposing materials, types of materials in contact, load, surface roughness, and time. The mechanical actions of attrition and abrasion may cause visible damage to prosthodontic restorative materials. Ceramics exhibit occlusal wear over time due to normal masticatory processes; however, the wear rate is generally acceptable (Seidel et al. 2020). Zirconia, one of the hardest dental restorative materials, is unlikely to wear and rather it is the opposing structures that are at risk. Fortunately, enamel and other restorative materials show favorable wear against polished zirconia (Janyavula et al. 2013, Passos et al. 2014, Amer et al. 2015, Zandparsa et al. 2016). It is difficult to repolish ceramic following adjustment (Tuncdemir et al. 2012), and when possible, it may be recommended to reglaze a clinically adjusted restoration (Ramadhan et al. 2018).
Artificial denture teeth used for fixed prosthodontic prostheses may exhibit more wear as compared with its use with removable prostheses. The wear rate is affected by the composition of the teeth, that is, conventional acrylic, composite, or a cross‐linked acrylic (Hao et al. 2014). Maintenance of polymeric restorative materials is expected because of a lack of wear resistance, and significant loss of occlusal surfaces may reduce masticatory efficiency and require replacement of worn components (Al‐Qarni and Goodacre 2021).
Because of their low hardness and high ductility, metallic dental alloys exhibit the least wear on opposing enamel when compared with other classes of materials (Branco et al. 2020).
5.2.13 Survival Rate
There are many studies in the peer‐reviewed literature that affirm the success of metal‐ceramic restorations; however, the database in terms of longevity for glass ceramics and polycrystalline ceramics is limited by comparison. Yet, the estimated five‐year survival rates for glass ceramic and zirconia single crowns are not significantly different from metal‐ceramic restorations (Sailer et al. 2015, Malament and Socransky 2010).
Polymeric restorative materials used as a replacement for missing hard and soft tissues in a fixed application will experience the most wear over time when compared with metals and ceramics (Al‐Qarni and Goodacre 2021, Priest et al. 2014, Hao et al. 2014).
CAD‐CAM technology has been useful for addressing factors responsible for controlling the fit of clinical restorations. Historically, goodness of fit of a final restoration was dependent upon a reduction in the accumulation of errors in a complex workflow from final impression to delivery of prosthesis (Samet et al. 2005). Sound biomechanical principles are of more importance than the materials selected for and integration of digital technology into practice, which can be successful when used in a proper manner. CAD‐CAM technology has been associated with an increase in quality and reproducibility and a standardized chain of production.
5.3 Manufacturing Considerations for CAD‐CAM Dental Materials
5.3.1 Subtractive Manufacturing of Dental Ceramics
5.3.1.1 Soft Milling
Machinability is generally defined as the ease with which a material can be cut, permitting the removal of materials with a satisfactory finish at a reasonable cost. An accurate and quantitative measurement of machinability is difficult, but various parameters such as tool wear, surface roughness, cutting force, cutting energy, drilling rates, brittleness, and tool penetration rate, are suggested as being related to the machinability of a certain material (Tsitrou et al. 2007, Spitznagel et al. 2018).
In general, the machinability of high‐strength ceramic materials is not as great as that of other dental materials. With a recent advancement in material science, physical properties of machinable dental ceramics have considerably improved. Improved strength is certainly a desirable characteristic, but this makes machining less predictable. Harder materials lead to greater tool wear, which negatively influence the quality of milled restorations. Diamond‐coated milling tools, with higher RPM, are commonly used for glass‐ceramic milling. This is commonly referred to as grinding instead of milling and inevitably induces surface damage. Although the use of lubricants/coolants during milling helps reduce surface stress, glass‐ceramic restorations are still more sensitive to surface flaws such as machining‐induced micro‐cracks due to their brittleness (Chen et al. 2020, Cho et al. 2019, Wu et al. 2019).
To overcome this limitation, a soft milling strategy is commonly used for high‐strength ceramic restorations. Lithium disilicate glass ceramic is a good example. Pre‐crystallized blocks, which are softer than fully crystallized ones, are used for milling to minimize potential problems such as surface cracks, margin chipping, and excessive tool wear. Once the restorations are successfully milled to the desired shape, pre‐crystallized ceramic restorations are heat‐treated to complete full crystallization to achieve their full mechanical strength and desired esthetic property.
The same strategy is commonly used to mill polycrystalline ceramics such as zirconia. Some zirconia blocks are fabricated to be milled in a fully sintered state, but most commercially available zirconia blocks are milled in a pre‐sintered state, often called a green state. Pre‐sintered zirconia is very soft, it is typically dry‐milled without adding a lubricant and metal milling tools without the diamond coating are used. Although most CAD‐CAM systems use dry‐milling for zirconia, some chairside systems still use wet‐milling for zirconia. Sintering zirconia causes a significant dimensional change. To compensate for this sintering shrinkage, zirconia restorations are milled larger than their actual size, then a volume shrinkage to actual size occurs through the sintering process. Most commercially available zirconia products undergo approximately 20% of sintering shrinkage, the exact value varies depending on products.
Not all ceramic blocks use the soft milling approach. Some ceramic blocks can be machined predictably in their final state. Examples of these ceramics include leucite‐reinforced glass ceramic, lithium aluminosilicate ceramic, and hybrid (composite‐ceramic) ceramics. Some other ceramic blocks, such as zirconia‐reinforced lithium silicate ceramics and zirconia ceramics, are available for both soft and hard milling (Figure 5.6a,b).
5.3.1.2 Margin Offset
Although the soft milling strategy reduces the incidence of surface cracks and defects, glass‐ceramic milling still has a high risk of developing unwanted flaws because of excessive stress applied on the brittle ceramic surface during milling. The area that these milling defects are most frequently seen is at the margin of restorations (Tsitrou et al. 2007). Micro chippings on the margin of milled ceramic restorations are more frequently seen when the margin design is thin and knife‐edged. As it is critical to achieve clinically acceptable marginal integrity, this limitation must be overcome. Many dental CAD systems use the strategy of setting the milling margin slightly away from the actual restoration margin. Offsetting the actual milling margin away from the restoration margin results in the restoration margin with some extra material around it (Figure 5.7a). About 100–200 μm of margin offset is commonly used for ceramic milling. While brittle and hard materials require a higher margin offset value, the optimal margin offset value varies depending on materials (Figure 5.8a,b). Although it is expected that margin chippings are reduced by adding more material around it as thin or knife‐edged margins are reinforced, this does not completely eliminate margin chipping issues. However, most micro chippings are limited within the extra‐material area and do not influence the actual restoration margin. After milling, the extra material around the margin is then manually removed using a gentle rotary instrument such as a diamond‐impregnated silicone disk (Figure 5.9a,b). It is recommended to use magnification for this procedure. Even when the restoration margin is milled without any notable chippings, removal of the extra material around the margin is still necessary to create an ideal contour and prevent overhangs (Figure 5.7b). If the margin chippings are extended to the actual restoration margin, the restoration can be re‐milled.

Figure 5.6 (a) Examples of hard milling ceramic blocks: from left to right, leucite‐reinforced glass ceramic, zirconia‐reinforced lithium silicate ceramic, and hybrid ceramic; (b) examples of soft milling ceramic blocks: from left to right, lithium disilicate ceramic, zirconia‐reinforced lithium silicate ceramic, and zirconia ceramic.
5.3.1.3 Milling Tools and Tool Diameter Compensation
Another challenge associated with ceramic milling is tool damage. Tool wear is affected by the type of material to be milled, with harder materials leading to greater tool wear (Cho et al. 2019). Even though use of a soft milling strategy allows ceramic restorations to be milled in a less hard stage, they are still harder than most other dental materials and have a higher risk of causing milling tool damage. For this reason, diamond‐coated milling tools are normally used for ceramic milling to protect the milling tools from accelerated wear or breakage. Damaged milling tools must be replaced to ensure quality of milled restorations and many milling machines provide a reliable milling tool monitoring system for the optimal management of milling tools (Figure 5.10). However, it is still necessary to examine milling tools before and after each milling cycle and replace them if significant damage was detected (Figure 5.11a,b).

Figure 5.7 (a) Milling margin is set 100~200 μm off from the actual restoration margin; (b) margin offset results in overhangs: extra material added on the margin must be manually removed after milling to prevent overhangs.
Use of milling tools with a smaller diameter may allow more precise milling, but it increases the risk of milling tool damage and may result in compromised milling quality and frequent milling tool replacement, which can increase the cost of dental milling. While milling tools with a 1 mm or greater diameter are commonly used to mill the intaglio surface of ceramic restorations, the shape and dimension of milling tools vary depending on the milling machine (Figure 5.12a–c). It is important for clinicians to know the shape and diameter of the milling tools as they limit surface morphology that can be generated as a result of milling. For example, if the diameter of the milling tool is 1 mm, negative shapes that are smaller than 1 mm cannot be accurately reproduced by using this milling tool. To compensate for this discrepancy and to fabricate dental restorations that can be fully seated, the intaglio surface of the restorations is modified by the CAD program before sending the data to a milling machine, which typically results in overmilling of inner sharp edges (Figure 5.13a). Tool diameter compensation, which refers to a feature of CAD software that adjusts the surface of a design to allow for the diameter of the milling tool, is necessary to fabricate dental restorations with acceptable seating, but at the same time, it can also cause a poor internal fit, which may result in compromised strength, retention, or esthetics (Figure 5.13b,c). Overmilling as a result of tool diameter compensation is commonly observed around cusp tips, occluso‐axial line angles, and irregular finish lines (Figure 5.14a). Preparation errors such as rough, spiked, or lipped finish lines, and complex tooth preparation forms, such as deep retentive grooves or deep occlusal morphology, are not well‐reproduced by milling (Renne et al. 2015, Renne et al. 2012
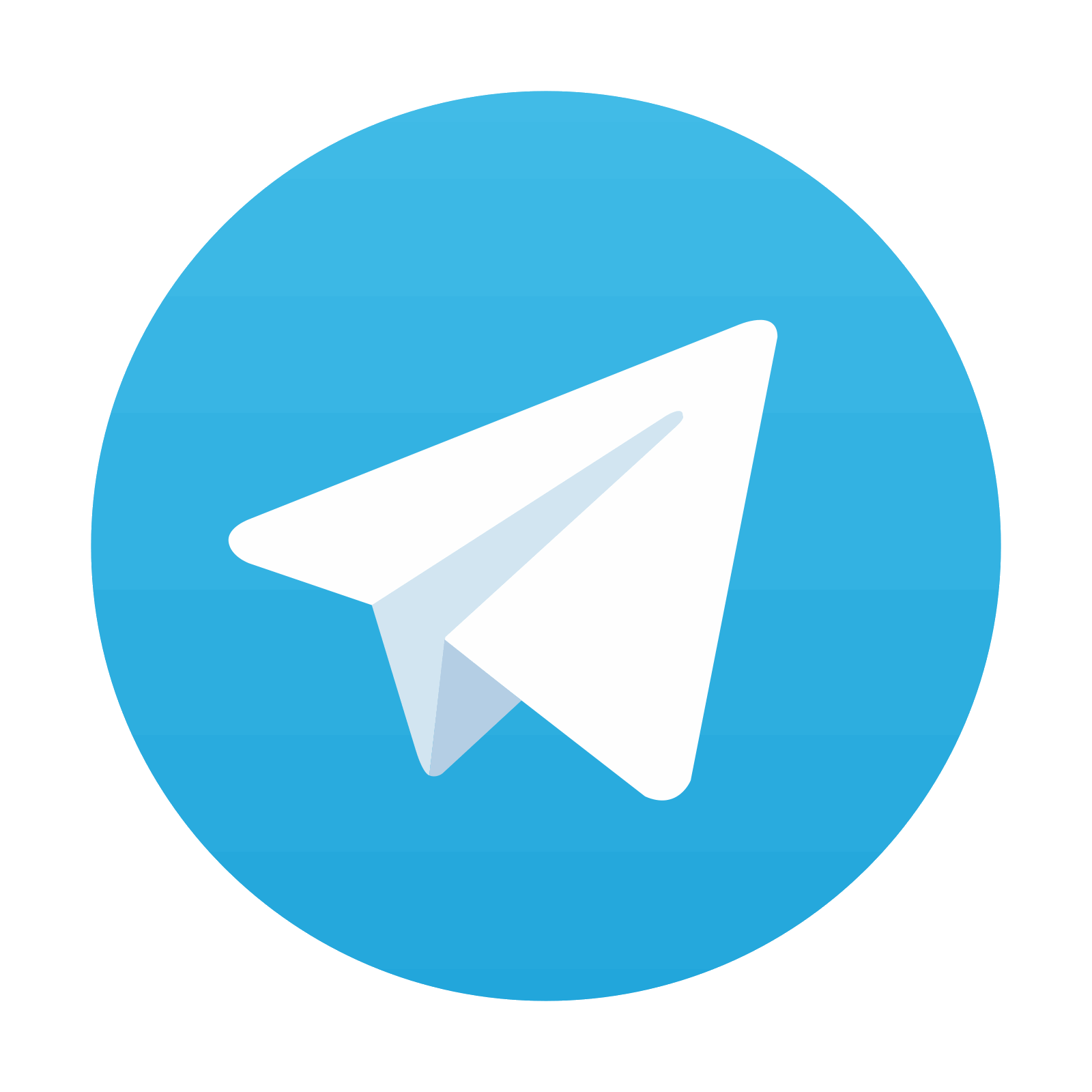
Stay updated, free dental videos. Join our Telegram channel

VIDEdental - Online dental courses
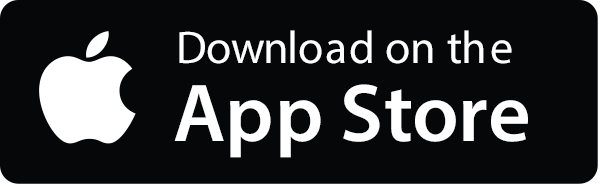
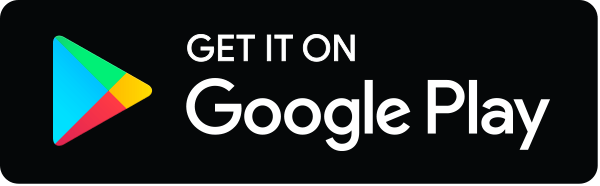