1
Digital Imaging
Jeffery B. Price
1.1 Introduction
Imaging, in one form or another, has been used in the dental profession since the first intraoral radiographic images were exposed by the German dentist, Otto Walkhoff (Langland et al. 1984) in early 1896, just 14 days after W.C. Röntgen publicly announced his discovery of X‐rays (McCoy 1919, Bushong 2008). Many landmark improvements have been made over the more than 120‐year history of oral radiography (American Association of Oral and Maxillofacial Radiologists (AAOMR) 2021, Molteni 2021).
The first receptors were glass, but film set the standard for the greater part of the twentieth century until the 1990s, when the development of digital radiography for dental use was commercialized by the Trophy company who released the RVGui system (Mouyen et al. 1989). Other companies such as Kodak, Gendex, Schick, Planmeca, Sirona, and Dexis were also early pioneers of digital radiography.
The adoption of digital radiography by the dental profession has been slow but steady and seems to have been governed, at least partly, by the “diffusion of innovation” theory espoused by Dr. Everett Rogers (2003). His work describes how various technological improvements have been adopted by end‐users of technology throughout the second‐half of the twentieth and early twenty‐first centuries. Two of the most important tenets of adoption of technology are the concepts of threshold and critical mass.
Threshold is a trait of a group and refers to the number of individuals in a group who must be using a technology or engaging in an activity before an individual adopts technology or engages in an activity. Critical mass is another characteristic of a group and occurs at the point in time when enough individuals in the group have adopted an innovation to allow for the self‐sustaining future growth of adoption of the innovation. As more innovators adopt a technology, such as digital radiography, the perceived benefit of the technology becomes greater and greater to ever increasing numbers of other future adopters until eventually the technology becomes commonplace.
Digital radiography is the most common advanced dental technology that patients experience during diagnostic visits. According to the most recent dental office survey completed by the Conference of Radiation Control Program Directors in 2014–2015 and published in 2019, 86% of offices used digital imaging (Conference of Radiation Control Program Directors 2019). The numbers of dentists using digital imaging continue to increase. If you are still using film, the question should not be “Should I switch to a digital radiography system?” but instead “Which digital system will most easily integrate into my office?”
This leads to another question – What advantages do digital radiography offer the dental profession as compared with simply continuing with the use of conventional film? Let us look at them.
1.1.1 Digital Versus Conventional Film Radiography
The most common speed class, or sensitivity, of intraoral film has been D‐speed film; the prime example of this film in the United States is Kodak’s Ultra‐Speed National Council on Radiation Protection and Measurements (NCRP 2012). The amount of radiation dose required to generate a diagnostic image using this film is approximately twice the amount required for Kodak’s Insight, an F‐speed film; in other words, F‐speed film is twice as fast as D‐speed film. According to Moyal, who used the 1999 NEXT data, the skin entrance dose of a typical D‐speed posterior bitewing is ~1.7 mGy (Moyal 2007). According to NCRP Report #172, the median skin entrance dose for a D‐speed film exposure is ~2.2 mGy, whereas the typical E–F‐speed film dose is ~1.3 mGy, and the median skin entrance dose from digital systems is ~0.8 mGy (NCRP 2012). According to NCRP #145 and others, it appears that dentists who use F‐speed film tend to over‐expose the film and then under‐develop it; this explains why the radiation dose savings with F‐speed film is not as great as it could be since F‐speed film is twice as fast as D‐speed film (NCRP 2004, NCRP 2012). If F‐speed film were used per the manufacturers’ instructions, the exposure time and/or milliamperage would be half that of D‐speed film and the radiation dose would then be half.
Why has there been so much resistance against the dental profession moving away from D‐speed film and embracing digital radiography? First of all, operating a dental office is much like running a fine‐tuned production or manufacturing facility; dentists spend years perfecting all the systems needed in a dental office, including the radiography system. Changing the type of imaging system risks upsetting the dentist’s capability to generate comprehensive diagnoses. To persuade individual dentists to change, there has to be compelling reasons. Until recently, most of the dentists in the United States have not been persuaded to make the change to digital radiography. It has taken many years to reach the threshold and the critical mass for the dental profession to make the switch to digital radiography. And, in all likelihood, there are dentists who will retire from active practice before they switch from film to digital radiography.
There are many reasons to adopt digital radiography: decreased environmental burdens by eliminating developer and fixer chemicals along with the associated silver and iodide bromide chemical waste; improved accuracy in image processing of digital images; decreased time required to capture and view images with increased efficiency of patient treatment; reduced radiation dose to the patient; improved ability to involve the patient in the diagnosis and treatment planning process with co‐diagnosis and patient education while viewing images on a computer monitor; and viewing software to dynamically enhance the image (Wenzel 2006, Wenzel and Møystad 2010, Farman et al. 2008). However, if dentists are to enjoy these benefits, the radiographic diagnoses for digital systems must be at least as reliably accurate as those obtained with film (Wenzel 2006).
Two primary co‐factors seem to be more important than others in driving more dentists away from film and toward digital radiography – the increased use of computers in the dental office and the reduced radiation doses with digital radiography. These factors will be explored further in the next section.
1.1.1.1 Increased Use of Computers in The Dental Office
This book’s focus is digital dentistry and later sections will deal with how computers interface with every facet of dentistry. The earliest uses of the computer in dentistry were in the business office and accounting. Over the ensuing years, computer use spread to full‐service practice management systems with digital electronic patient charts, including digital image management systems. The use of computers in the business operations side of the dental practice allowed dentists to gain experience and confidence in how computers could increase financial efficiency and reliability in practice operations. The next step was to integrate computer applications developed for clinical uses. As a component of creating the virtual dental patient, initially, the two most prominent roles were electronic patient records and digital radiography. In the following sections, we will explore the attributes of digital radiography, including decreased radiation doses as compared with film, improved operator workflow and efficiency, fewer errors with fewer retakes, wider dynamic range, increased opportunity for co‐diagnosis and patient education with the patient, improved image storage and retrievability, and communication with other providers (Farman et al. 2008, Wenzel and Møystad 2010). The virtual dental patient will be explored in later chapters.
1.1.1.2 Review of Basic Terminology
Conventional intraoral film technology, such as periapical and bitewing imaging, uses the direct exposure technique whereby X‐ray photons directly stimulate silver bromide crystals to create a latent image. Today’s direct digital X‐ray sensor refers most commonly to a complementary metal oxide semi‐conductor (CMOS) sensor that is directly cabled into the computer via a USB port. At the time of the exposure, X‐ray photons are detected by cesium iodide or perhaps gadolinium oxide scintillators within the sensor, which then emits light photons. These light photons are then detected within the sensor pixel by pixel, which allows for almost instantaneous image formation on the computer monitor. Most clinicians view this instantaneous image display as the most advantageous characteristic of direct digital imaging.
The other choice for digital radiography today is an indirect digital technique known as photostimulable phosphor, or PSP plates; these plates resemble conventional film in appearance and clinical handling. During exposure, the latent image is captured within energetic phosphor electrons; during processing, the energetic phosphors are stimulated by a red laser light beam; the latent energy stored in the phosphor electrons is released as a green light, which is captured, processed, and, finally, digitally manipulated by the computer’s graphic card into images relayed to the computer’s display. The “indirect” term refers to the extra processing step of the plates as compared with the direct method when using the CMOS sensor. The most attractive aspect of PSP may be that the clinical handling of the phosphor plates is exactly like handling film; therefore, most offices find the transition from film to PSP to be very manageable and user‐friendly.
Panoramic imaging commonly uses direct digital techniques as well. The panoramic X‐ray beam is collimated to a slit; therefore, the direct digital sensor is several pixels wide and continually captures the signal of the remnant X‐ray beam as the panoramic X‐ray source/sensor assembly continually moves around the patient’s head. The path of the source/sensor assembly is the same whether the receptor is an indirect film, PSP, or direct digital system. Clinicians who are using direct digital receptors generally opt for a direct digital panoramic system to avoid the need to purchase a PSP processor for the panoramic system.
Orthodontists require a cephalometric system and when moving from film to digital, again have two choices: direct digital and indirect digital. The larger flat panel digital receptor systems provide an instantaneous image but are slightly more costly than the indirect PSP systems; however, the direct digital systems obviate the need to purchase and maintain PSP processors. The higher the volume of patients in the office, the quicker the financial payback for the direct digital machine.
1.1.1.3 Image Quality Comparison between Direct and Indirect Digital Radiography
Some dentists decide on which system to purchase solely based on the speed of the system, with the direct digital system being the fastest. Another important factor to consider is the perpetual question of image quality. Perhaps the better question to ask may be, “Is there a significant difference between the diagnostic capability of direct and indirect digital radiography systems?” One of the primary diagnostic tasks facing dentists on a daily basis is caries diagnosis. There are several studies that have evaluated the efficacy of the two systems at this common task. The answer is that there is no difference between the two systems in diagnostic efficacy – both direct digital and indirect digital with PSP plates will provide comparable diagnostic capability for caries detection, in modern systems (Wenzel et al. 2007, Berkhout et al. 2007, Li et al. 2007).
One important consideration when comparing systems is to ensure the images have the same bit depth . Bit depth refers to the numbers of shades of gray used to generate the image and are expressed exponentially in Table 1.1.
Table 1.1 Bit depth table correlating the relationship of the exponential increase in the number of shades of gray available in radiographic images as the bit depth increases.
Bit depth | Expression | Number of shades of gray |
---|---|---|
1 | 21 | 2 |
2 | 22 | 4 |
3 | 23 | 8 |
4 | 24 | 16 |
5 | 25 | 32 |
6 | 26 | 64 |
7 | 27 | 128 |
8 | 28 | 256 |
9 | 29 | 512 |
10 | 210 | 1 024 |
11 | 211 | 2 048 |
12 | 212 | 4 096 |
13 | 213 | 8 192 |
14 | 214 | 16 384 |
15 | 215 | 32 768 |
16 | 216 | 65 536 |
The early digital systems had a bit depth of 8 with 256 shades of gray, which may seem adequate since the human eye can only detect approximately 20–30 shades of gray at any one time in any one image. Most digital systems today generate images at 12 or even 16 bit depth, i.e. images that have 4 096–65 536 shades of gray; therefore, 12, 14, or 16 bit depth images provide for greater contrast resolution (Bushberg et al. 2012). Proper image processing is a skill that must be learned to fully utilize all of the information contained in today’s digital images. Conventional film systems do not have discrete shades of gray; rather, film systems produce analog images with the number of shades of gray limited only by the number of silver ions activated in each cluster of silver atoms in the latent image within the silver halide lattice of the film emulsion. Therefore, when comparing digital systems, one should ensure that the bit depth of the systems is comparable, and remember that, over time, the higher bit depth systems will require larger computer storage capacities due to the larger file sizes associated with the increased amount of digital information requirements of the higher bit depth images.
1.1.1.4 Amount of Radiation Required to Use Direct and Indirect Digital Radiography
One other factor that dentists should consider when evaluating which system to use is how much radiation is required for each system to generate a diagnostic image. To determine the answer to this question, clinicians should be familiar with the term dynamic range , which refers to the performance of a radiographic receptor system in relation to the amount of radiation required to produce a desired amount of optical density within the image. The Hurter and Driffield (H&D) characteristic curve chart was initially developed for use with film systems and can also be used with direct digital and indirect digital systems (Bushong 2008, Bushberg et al. 2012). The indirect digital system with PSP plates has the widest dynamic range, even wider than film, which means that PSP plates are more sensitive to lower levels of radiation than either conventional film or direct digital CMOS detectors. At the upper range of diagnostic exposures, the PSP plates do not experience burnout as quickly as film or direct digital until very high radiation doses are delivered. Thus, PSP systems can handle a wider range of radiation dose and still deliver a diagnostic image, which may be a good feature. However, dentists should be aware that the operator of the equipment may be delivering higher radiation doses than are necessary simply because their radiographic system has not been properly calibrated (Bushong 2008, Bushberg et al. 2012, Huda et al. 1997, Hildebolt et al. 2000). When using PSP imaging, it is very important to calibrate your technique factors of kV, mA, and time to the lowest settings that yield diagnostic images to avoid over‐irradiating your patients.
1.1.2 Radiation Safety of Diagnostic Radiography
There are several principles of radiation safety: as low as reasonably achievable (ALARA); justification, limitation, optimization, and the use of selection criteria. These factors will be briefly reviewed and then how digital radiography plays a vital role in the improved safety of modern radiography will be discussed.
The acronym ALARA stands for As Low As Reasonably Achievable , and, in reality, is very straightforward. In the dental profession, dentists, and dental auxiliaries are required to use medically accepted radiation safety techniques that keep radiation doses low and do not cause an undue burden on the operator or clinician. An example from NCRP Report #177 Recommendation #31, 6.1.1, states “Image receptors of speeds slower than ANSI Speed Group E/F shall not be used for intraoral radiography (i.e. D‐speed film shall not be used)” (NCRP 2019). This means that offices do not have to switch to digital but rather could switch to E‐ or F‐speed film but must switch to at least E‐speed film to be in compliance with this report.
When clinicians go through the process of examining patients and formulating diagnostic questions, they are justifying the radiographic examination. This principle of justification is one of the primary principles of radiation safety. With digital radiography, radiation doses are very low; so low in fact, that if there is a diagnostic question that can only be answered with the information obtained from a dental radiograph, the risk from the radiograph is low enough that the “risk to benefit analysis” is always in favor of exposing the radiograph. Another way to consider the question is whether the treatment outcome will benefit from the information obtained from the radiograph. If the treatment outcome will be improved, then order and expose the radiograph. If you cannot determine how the treatment outcome will be improved by the radiograph under consideration, the radiographic examination is not indicated.
The principle of limitation means that the radiographer is doing everything possible to limit the radiation dose to the patient. Limitation includes decreasing the numbers of radiographic exposures and limiting the amount of radiation used for exposures. Decreasing the numbers of exposures is best achieved by following selection criteria guidelines such as those published in 2012 by the American Dental Association (ADA) and FDA. These guidelines give the dentist several common scenarios that are seen in practice and offer suggestions on which radiographs may be appropriate. This publication provides an excellent review of the topic and is best summarized by a sentence found in its conclusion: “Radiographs should be taken only when there is an expectation that the diagnostic yield will affect patient care” (ADA and FDA 2012). Limiting the actual size of the X‐ray beam by use of rectangular collimation reduces the radiation dose to the patient by ~40–60% and has been a standard recommendation for intraoral dental radiography by the NCRP, the ADA, and other organizations for many years (NCRP 2019, ADA 2006, NCRP 2004, Johnson et al. 2014, Shetty et al. 2019, Lurie 2019). In panoramic imaging, the X‐ray beam is collimated to a slit shape, whereas in cone beam CT, the X‐ray beam has a cone shape. The purpose of collimating the primary X‐ray beam to these shapes is to limit patient radiation doses.
How does digital radiography assist with managing radiation safety? As mentioned earlier, digital receptors require less radiation dose than film receptors. In the 2012 NCRP Report #172, Section 6.4.1.3, it was recommended that dentists adopt a diagnostic reference level (DRL) for intraoral radiographs of 1.2 mGy. This dose is the median dose for E‐ and F‐speed film systems and is higher than the dose for digital systems but lower than the dose for D‐speed film. This means that to predictably achieve this ambitious goal, dentists who are still using D‐speed film will need to either switch to F‐speed film or transition to a digital system (NCRP 2012). The expectation is that practitioners contemplating a change in technique will transition to digital radiography.
1.1.2.1 Radiation Dosimetry
The dental profession owns more X‐ray machines than any other profession. This translates into dentists exposing patients to a lot of radiographs. The doses are very small, but the patients expect answers to their questions about the safety of the radiographs that are being recommended, and, it is part of the dentist’s professional responsibility to the patients.
The international system uses the Gray (Gy) or milliGray (mGy) and microGray (μGy) to describe the amount of radiation dose that is absorbed by the patient’s skin (skin entrance dose) or by their internal organs. This dose is measured by devices such as ionization chambers or optically stimulated dosimeters (OSLs). There are different types of tissues in our body and they all have a different response or sensitivity to radiation. For instance, the child’s thyroid gland seems to be the most sensitive tissue that is in the path of our X‐ray beams while the mature mandibular nerve may be the least sensitive tissue type in the maxillofacial region (Hall and Giaccia 2012). Of course, dentists only deal with diagnostic radiation, but there are other types of radiation such as gamma rays, alpha particles, and beta particles. To provide a way to measure the effect on the body’s various tissues when exposed by radiation from the various sources, a term known as equivalent dose is used. This term is expressed in Sieverts (S) or milliSieverts (mSv) and microSieverts (μSv). Finally, another term known as effective dose is used to compare the risk of radiographic examinations. This is the most important term for dental professionals to be familiar with since this is the term that accounts for the type of radiation used (diagnostic in our case); the type and volume of tissues exposed by the X‐ray beam in the exam, whether it is a bitewing, a panoramic, a cone beam CT, or a chest X‐ray and the radiation sensitivity of these tissues. Using this term is like comparing apples with apples. By using this term, the health risk of a panoramic radiograph can be compared with the risk of an abdominal CT or a head CT, for example, as the risk of these radiographic examinations can all be measured using effective dose.
When patients ask us about how safe a particular radiographic examination may be, they are really asking whether that X‐ray is going to cause cancer and whether that cancer will increase their chance of dying. When medical physicists estimate the risk of X‐rays in describing effective dose as measured in Sieverts and microSieverts for dentistry, they are referring to the risk of developing a fatal cancer. The risk is usually given as the rate of excess cancers per million. To accurately judge this number, the clinician really needs to know the background rate of cancer (and fatal cancer) in the population. According to the American Cancer Society, the average person living in the United States has an approximately 40% chance of developing cancer during his or her lifetime. Moreover, the rate of fatality of this affected group is 50%; therefore, the overall fatal cancer rate in the United States is 20%, or 200 000 per million people (Siegel et al. 2021). Now, when you read in the radiation dosimetry table (Table 1.2) that if a million people had a panoramic exposure and the excess cancer rate in those one million people was 0.9 per million, you would know that the total cancer rate changed from 200 000 to 200 000.9 per million. On a percentage basis, that is a very small percentage indeed – a 0.00045% excess risk of developing cancer. Of course, these are population‐based numbers and are the best estimates groups like the NCRP can derive. Despite the best efforts of many researchers in the field of radiation biology, we do not yet know with certainty how the human cell responds and interacts with low‐dose ionizing radiation, particularly at the very low doses of ionizing radiation seen in most dental radiographic examinations. To be safe and err on the side of caution, which is the prudent course of action, it is assumed that some cellular and some genetic damage is possible in accordance with a dose‐response model known as the linear non‐threshold model of radiation interaction, which is based on the assumption that in the low‐dose range of radiation exposures, any radiation dose will increase the risk of excess cancer and/or heritable disease in a simple proportionate manner (Hall and Giaccia 2012, Lurie 2019).
Table 1.2 Excess fatal cancer risk from various dental radiographic examinations.
Source: permission granted by Dr. John Ludlow.
Effective doses from dental and maxillofacial X‐ray techniques and probability of excess fatal cancer risk per million examinations | |||
---|---|---|---|
Technique | Dose (mSv) | CA risk per million exams | Background equivalency |
Panoramic – indirect digital | 16 | 0.9 | 2 d |
Skull/cephalometrics – indirect digital | 5 | 0.3 | 17 h |
FMX (PSP or F‐speed film – rectangular collimation) | 35 | 2 | 4.3 d |
FMX (PSP or F‐speed film – round collimation) | 171 | 9 | 21 d |
FMX (D‐speed film – round collimation) | 388 | 21 | 47 d |
Single PA or bitewing (PSP or F‐speed film – rectangular collimation) | 1.25 | 0.1 | 3.6 h |
Single PA or bitewing (PSP or F‐speed film – round collimation) | 9.5 | 0.5 | 1 d |
Single PA or bitewing (D‐speed film – round collimation) | 22 | 1.2 | 2.6 d |
4 Bitewings (PSP or F‐speed film – rectangular collimation) | 5 | 0.3 | 17 h |
4 Bitewings (PSP or F‐speed film – round collimation) | 38 | 2 | 4 h |
4 Bitewings (D‐speed film – rectangular collimation) | 88 | 5.5 | 11 h |
Conventional tomogram (8 × 8 cm field of view) | 10 | 0.5 | 1 d |
Cone beam CT exam (Carestream 9300 10×10 cm full jaw) | 79 | 5 | 10 d |
Cone beam CT exam (Carestream 9300 5 ×5 cm, posterior mandible) | 46 | 3 | 6 d |
Cone beam CT exam (Sirona Galileos) | 70 | 4 | 8 d |
Maxillo‐mandibular MDCT | 2100 | 153 | 256 d |
Table 1.2 is used with the permission of Dr. John Ludlow who has accomplished a great deal of radiation dosimetry research in the oral and maxillofacial region. There is one more column that needs some explanation – background equivalency. In the United States, the average person receives ~8 μSv of effective dose of ionizing radiation per day (NCRP 2009). Take a look at the first examination – a panoramic exposure: it has an effective dose of ~16 μSv; if you divide 16 by 8 μSv per day, the result is two days of background equivalency. Using this method, you now know that the amount of effective dose in the average panoramic examination equals the same amount of radiation that the average person receives over the course of two days. This same exercise has been completed for the examinations listed in the table. For examinations not listed, you can calculate the background equivalency by following the simple calculations above. The intended use of effective dose is to compare population risks; however, this use as described above is a quick and easy patient education tool that most of our patients can quickly understand.
1.1.3 Uses of Two‐Dimensional (2D) Systems in Daily Practice
The use of standard intraoral and extraoral imaging for clinical dentistry has been available for many years and includes caries and periodontal diagnosis, endodontic diagnosis, detection, and evaluation of oral and maxillofacial pathology and evaluation of craniofacial developmental disorders.
1.1.3.1 Caries Diagnosis
The truth is that diagnosing early carious lesions with bitewing radiographs is much more difficult than it appears to be at first impression. In most studies, researchers have found that a predictably accurate caries diagnosis rate of 60% would be deemed acceptable. In a 2002 study, Mileman and Van Den Hout compared the ability of Dutch dental students and practicing general dentists to diagnose dentinal caries on radiographs. The students performed almost as well as experienced dentists (Mileman and Van Den Hout 2002, Bader et al. 2001, Bader et al. 2002, Dove 2001). Caries diagnosis and how modern methods of caries diagnosis are changing the paradigm from the past methods of diagnosing caries will be explored next.
Caries detection is a basic task that all dentists are taught in dental school. In principle, it is very simple – detect mineral loss in teeth visually, radiographically, or by some other adjunctive method. There can be many issues that affect this task, including training, experience, and subjectivity of the observer; operating conditions, and reliability of the diagnostic equipment. Accordingly, these factors and others can all act in concert and often, the end result is that this “simple” task becomes complex. It is important to realize that the diagnosis of a carious lesion is only one aspect of the entire management phase for dental caries. In fact, there are many aspects of managing the caries process besides diagnosis. The lesion needs to be assessed as to whether the caries is limited to enamel or if it has progressed into dentin. A determination of whether the lesion is cavitated needs to be established since cavitated lesions continue to trap bacterial plaque and will need to be restored. The activity level of the lesion needs to be determined; a single evaluation will only tell the clinician the condition of the tooth at that single point in time; the demineralization rate may be increasing or, perhaps it is decreasing; larger lesions will not require a detailed evaluation of activity, but smaller lesions will need this level of examination and follow‐up. Finally, the therapeutic or operative management options for the lesion need to be considered based on these previous findings.
One thing to keep in mind is that most of the past research on caries detection has focused on occlusal and smooth surface caries. There are two reasons for this – first of all, from a population standpoint, more new carious lesions are occlusal lesions today than in the past (NIH 2001, Zandona et al. 2012, Marthaler 2004, Pitts 2009, Zandona and Longbottom 2019), and secondly, many studies rely on screening examinations without intraoral radiographic capability (Bader et al. 2001, Zero 1999). Let’s look at the traditional classification system that U.S. dentists have used in the past and a system that is being taught in many schools today.
1.1.3.2 Caries Classifications
The standard ADA caries classification system was updated in 2015 and designated dental caries as initial, moderate, and advanced (see Figure 1.1) (Young et al. 2015). There have been many attempts over the years to develop one universal caries classification system that clinical dentists and research dentists can use in the United States and internationally. As the result of the International Consensus Workshop on Caries Clinical Trials (ICW‐CCT) held in 2002, the work on the International Caries Detection and Assessment System (ICDAS) (n.d.) was begun in earnest, and has now merged into the International Caries Classification and Management System (ICCMS) (n.d.) as the leading international system for caries diagnosis (Ismail et al. 2007, ICDAS, ICCMS). The ICDAS for caries diagnosis offers a six‐stage, visual‐based system for detection and assessment of coronal caries. It has been thoroughly tested and has been found to be both clinically reliable and predictable. Perhaps its greatest strengths are that it is evidence based, combining features from several previously existing systems and does not rely on surface cavitation before caries can be diagnosed. In addition, a very detailed and objective radiographic rating system is included (Figures 1.2–1.4). Many previous systems relied on conflicting levels of disease activity before a diagnosis of caries, but with the ICCMS/ICDAS system, leading cariologists have been able to standardize definitions and levels of the disease process. The ICCMS/ICDAS evaluation system appears to be the acceptable standard for caries diagnosis internationally and is gaining acceptance in the United States (Mitchell et al. 2017, Zandona and Longbottom 2019).
1.1.3.3 Ethics of Caries Diagnosis
One of the five principles of the ADA’s Code of Ethics is nonmaleficence, which states that dentists should “do no harm” to his or her patients (ADA 2020). By enhancing their caries detection skills, dental practitioners can detect areas of demineralization and caries at the earliest possible stages; these teeth can then be managed with fluorides and other conservative therapies (Bravo et al. 1997, Marinho et al. 2003, Petersson et al. 2005). This scenario for managing teeth with early caries will hopefully make some inroads into the decades‐old practice of restoring small demineralized areas because they are going to need fillings anyway and you might as well fill them now instead of waiting until they get bigger (Baelum et al. 2006). Continuing to stress the preventive approach to managing early caries begins with early diagnosis. What better way to “do no harm” to our patients than to avoid placing restorations in these teeth with early demineralized enamel lesions and remineralize them instead?

Figure 1.1 ADA caries classification system.

Figure 1.2 ICDAS caries classification system.

Figure 1.3 ICDAS clinical examples.
1.1.4 Non‐Radiographic Methods of Caries Diagnosis
1.1.4.1 Quantitative Light‐Induced Fluorescence
It has been shown that tooth enamel has a natural fluorescence by using a CCD‐based intraoral camera with specially developed software for image capture and storage quantitative light‐induced fluorescence (QLF) Patient, Inspektor Research Systems BV, Amsterdam, The Netherlands. QLF technology measures (quantifies) the refractive differences between healthy enamel and demineralized, porous enamel with areas of caries and demineralization showing less fluorescence. With the use of a fluorescent dye, which can be applied to dentin, the QLF system can also be used to detect dentinal lesions in addition to enamel lesions. A major advantage of the QLF system is that these changes in tooth mineralization levels can be tracked over time using the documented measurements of fluorescence and the images from the intraoral camera. The third generation Qraycam system has been shown to produce markedly improved results at caries detection when compared with earlier models (Angmar‐Månsson and Ten Bosch 2001, Pretty and Maupome 2004, Amaechi and Higham 2002, Pretty 2006, Park et al. 2019).

Figure 1.4 ICDAS radiographic scoring system.
1.1.4.2 Laser Fluorescence
The DIAGNOdent uses laser fluorescence for caries detection, a technique that relies on the differential refraction of light as it passes through sound tooth structure versus carious tooth structure. As described by Lussi et al. in 2004, a 650 nm light beam, which is in the red spectrum of visible light, is introduced onto the region of interest on the tooth via a tip containing a laser diode. As part of the same tip, there is an optical fiber that collects reflected light and transmits it to a photo diode with a filter to remove the higher‐frequency light wavelengths, leaving only the lower‐frequency fluorescent light that was emitted by the reaction with the suspected carious lesion. This light is then measured or quantified, hence the name “quantified laser fluorescence.” One potential drawback with the DIAGNOdent is the increased incidence of false‐positive readings in the presence of stained fissures, plaque and calculus, prophy paste, existing pit and fissure sealants, and existing restorative materials. A review of caries detection technologies published in the Journal of Dentistry in 2006 by Pretty that compared the DIAGNOdent technology with other caries detection technologies such as Electronic Caries Monitor (ECM), FOTI, and QLF showed that the DIAGNOdent technology had an extremely high specificity or ability to detect caries (Lussi et al. 2004, Tranaeus et al. 2005, Côrtes et al. 2003, Lussi et al. 1999, Pretty 2006).
1.1.4.3 Electrical Conductance
The basic concept behind electrical conductance technology is that there is a differential conductivity between sound versus demineralized tooth enamel due to changes in porosity; saliva soaks into the pores of the demineralized enamel and increases the electrical conductivity of the tooth.
There has been a long‐standing interest in using electrical conductance for caries detection; original work on this concept was published as early as 1956 by Mumford. One of the first modern devices was the ECM, which was a fixed‐frequency device used in the 1990s. The clinical success of the ECM was mixed as evidenced by the lack of reliable diagnostic predictability (Amaechi 2009, Mumford 1956, Tranaeus et al. 2005).
1.1.4.4 Alternating Current Impedance Spectroscopy
The CarieScan device uses multiple electrical frequencies (alternating current impedance spectroscopy) to detect and diagnose occlusal and smooth surface caries. By using compressed air to keep the tooth saliva‐free, one specific area on a tooth can be isolated from the remaining areas and can be examined. If an entire surface needs to be evaluated, an electrolyte solution is introduced and the tip of the probe is placed over the larger area. The diagnostic reliability of this device is more accurate and reliable than ECM, and, according to the literature, stains and discolorations do not interfere with the proper use of the device. It appears to have good potential as a caries detection technology (Tranaeus et al. 2005, Amaechi 2009, Pitts et al. 2007, Pitts 2010).
1.1.4.5 Frequency‐Domain Laser‐Induced Infrared Photothermal Radiometry and Modulated Luminescence (PTR/LUM)
This technology is known as the Canary system (Quantum Dental Technologies, Inc., Toronto, CA). It relies on the absorption of infrared laser light by the tooth with measurement of the subsequent temperature change, which is in the 1 °C range. This optical to thermal energy conversion is able to transmit highly accurate information regarding tooth densities at greater depths than visual‐only techniques. Early laboratory testing showed better sensitivity for caries detection for this technology than for radiography, visual, or for DIAGNOdent technology and further studies continue to show satisfactory performance (FDA 2012, Amaechi 2009, Jeon et al. 2007, Jeon et al. 2010, Sivagurunathan et al. 2010, Matvienko et al. 2011, Abrams et al. 2011, Xing et al. 2019).
1.1.5 Dental Cone Beam Computed Tomography
Cone Beam Computed Tomography (CBCT) is arguably the most exciting advancement in oral radiology since panoramic radiology in the 1950s and 1960s, and perhaps since Roentgen’s discovery of X‐rays in 1895 (Mozzo et al. 1998). The concept of using a cone‐shaped X‐ray beam to generate three‐dimensional (3D) images has been successfully used in vascular imaging since the 1980s (Bushberg et al. 2012), and after many iterations, is now used in dentistry. Many textbooks offer in‐depth explanations of the technical features of CBCT (White and Pharoah 2014, Miles 2012, Sarment 2014, Brown 2013, Zoller and Neugebauer 2008). Therefore, only a summary is provided here using a full maxillofacial field of view CBCT as an example.
While the X‐ray source is rotating around the patient, most manufacturers today design the electrical circuit to pulse the source on and off approximately 15 times per second; the best analogy to use is that the computer is receiving the equivalent of a low‐dose X‐ray movie at about 15 frames per second quality. At the end of the image acquisition phase for most systems, the reconstruction computer then has anywhere from 200 to 500 basis or projection images, depending on the resolution of the scan. These images are then processed using any one of several algorithms. The original, classic algorithm is the back projection reconstruction algorithm that was a key element of the work of Sir Godfrey Hounsfield and Allan McCormack who shared the Nobel Prize in Medicine in 1979 (Bushberg et al. 2012). Many other algorithms such as the Feldkamp algorithm, the cone beam algorithm, the iterative algorithm, and others are used in various forms today as well as metal artifact reduction algorithms. Manufacturers have their own proprietary algorithms that are applied to CBCT volumes as well. The end result of the processing is not only 3D volumes, but also multi‐planar reconstructed (MPR) images that can be evaluated in the following three standard planes of axial, coronal, and sagittal images (Figures 1.5
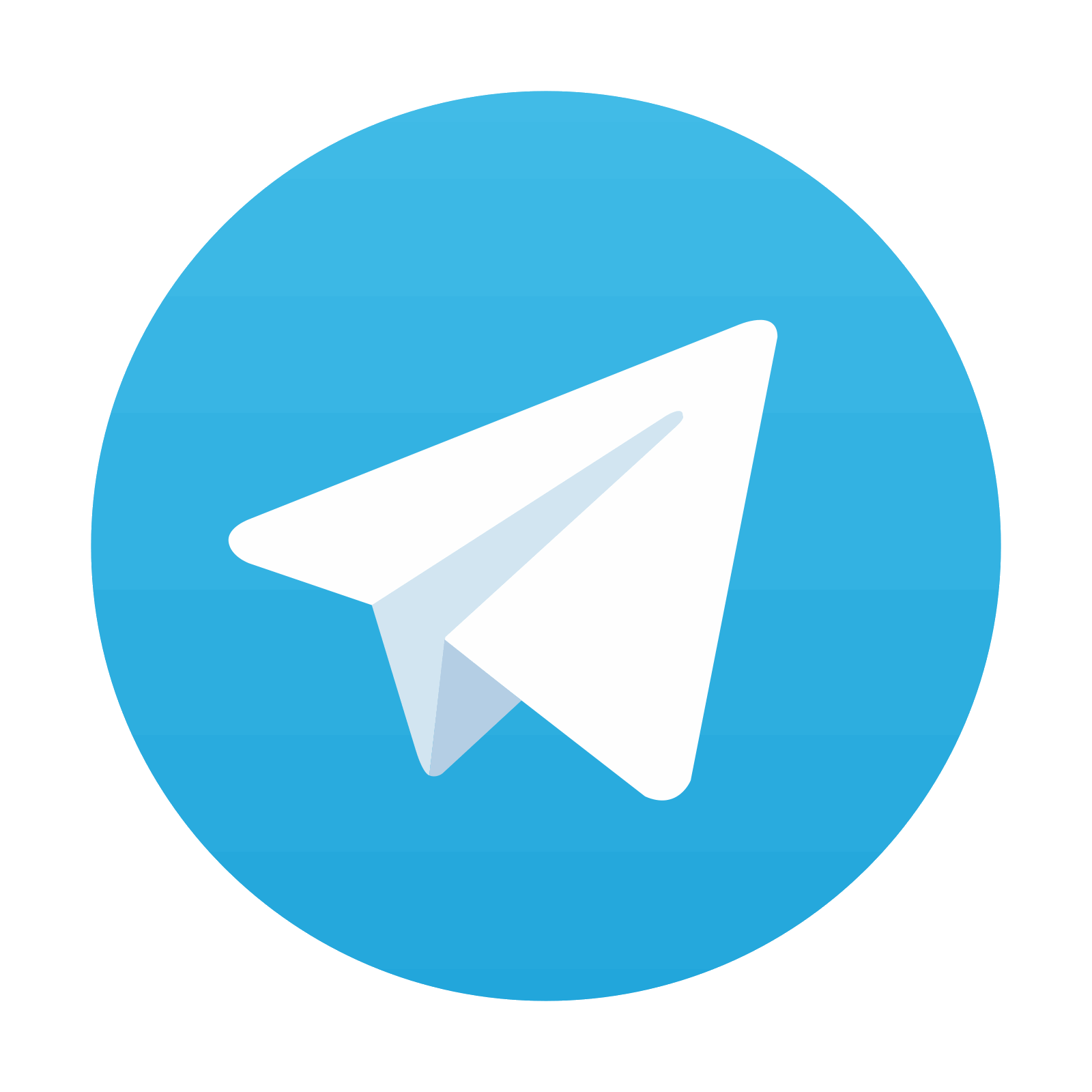
Stay updated, free dental videos. Join our Telegram channel

VIDEdental - Online dental courses
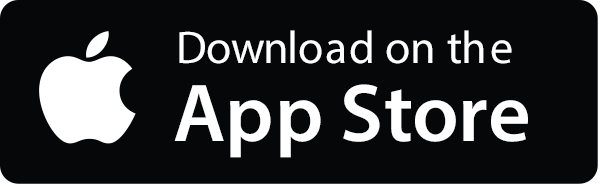
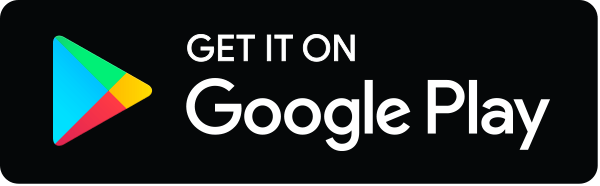