4
Additive Manufacturing Procedures and Clinical Applications in Restorative Dentistry
Marta Revilla-León and Amirali Zandinejad
4.1 Introduction
Additive manufacturing (AM) technologies build physical three‐dimensional (3D) geometries by a consecutive layer‐by‐layer addition of material (ISO:17296‐2:2015, ISO:52900). During the growth of AM procedures, there has been plentiful terms and definitions, frequently with reference to explicit application areas and trademarks. This is frequently vague and unclear, which hampers communication (ISO:52900). The International Organization for Standardization (ISO) in collaboration with the American Society for Testing and Materials (ASTM) Committee F42 aim to create a common set of ISO/ASTM standards on AM, resulting in more than 19 published ISO standards today, and signifies the best reference for AM terminology (ISO/TC: 261).
Additive manufacturing technologies can also produce 3D structures that can actively change their properties under environmental influences. These AM procedures are normally called four‐dimensional (4D) AM (Gladman et al. 2016). However, in dentistry, there are no applications yet analyzed for 4D AM procedures.
The capability to manufacture complex geometries is one of the most remarkable advantages among the different manufacturing benefits that AM techniques provide compared with subtractive methods (Figure 4.1) (Wieding et al. 2015, Spece et al. 2021). Furthermore, different AM technologies have been investigated to process different materials at the same time, which have the potential to introduce multi‐functionality on additively manufactured devices (Wang et al. 2016, Bandyopadhyay and Heer 2018, Whitehead and Lipson 2020). Previous authors have described a reduction in manufacturing costs, due to minimized material waste, elimination of production steps and decreased fabrication time for AM methods compared with milling techniques (Thomas and Gilbert 2014); however, there is no study available that compares the manufacturing cost and effectiveness in the dental field.
Technology maturation is a fundamental element for clinical development and manufacturing standards implementation. Interestingly, the applications of AM technologies in the dental field have increased 35% from 2017 to 2018, with an expected increase in market size of $9.5 billion by 2027 (Stephenson 2015, Ugalmugle 2018). This promising expected growth will facilitate the improvement and development of AM technologies for dental applications. Furthermore, the dental literature evaluating the applications of AM technologies in the dental field have also significantly increased since 2009 (Javavid and Haleem 2019). The future applications of AM technologies in dentistry are promising.
Removable and fixed dental prostheses (FDPs) fabricated using conventional methods such as casting and milling techniques have been analyzed, reporting adequate long‐term clinical success and survival rates (Harder and Kern 2009, Kapos and Evans 2014, Patzelt et al. 2015, Pjetursson et al. 2012, Pjetursson et al. 2018, Sailer et al. 2018). Additive manufacturing techniques incorporate a new manufacturing method that provides fabricating advantages when compared with our current manufacturing gold standards, such as casting and milling methods; however, for a standardization producing implementation, the mechanical properties, biocompatibility, and clinical performance of AM polymeric, metal, and ceramic dental prostheses need to be analyzed to justify its clinical use.
4.2 Manufacturing Workflow and Manufacturing Accuracy
When using subtractive or additive fabricating methods or computer‐aided design and computer‐aided manufacturing (CAD‐CAM) procedures, the manufacturing workflow of a dental device starts with its virtual design, normally obtained using a dental or non‐dental CAD software program (Witkowski 2005). The virtual design is contained typically in a digital standard tessellation language (STL) file that represents the surface geometry of the dental device (3D Systems 1988). The geometry is encoded as a “polygon soup,” i.e. a list of polygons in a 3D space that define the discrete approximation (or the mesh model) of the underlying true geometry.
The STL file is then used to manufacture the virtual design of the dental device using computer‐aided AM technologies (Witkowski 2005, Singh 2013). The manufacturing process can be divided into three steps, namely: data processing, manufacturing, and post‐processing procedures (Witkowski 2005, Singh 2013).

Figure 4.1 Additively manufactured metal complex geometry.
- Data processing comprises the specification of the printing parameters, determination of the support parameters, and the slicing of the virtual design contained on the STL file using the CAM software program of the selected printer. The printing parameters are dependent on the material, AM technology, and selected 3D printer.
- Manufacturing involves the AM of the dental device using the selected 3D printer.
- Post‐processing procedures comprise the process’s need to complete the finishing of the AM dental device. The post‐processing methods are dependent on the material and the technology selected to manufacture the device (Decker 1998, Taormina et al. 2018).
The manufacturing accuracy of an AM technology depends on the manufacturing trinomial, namely AM technology, printer, and material selected. The accuracy will be the result of the accumulation of distortion of each manufacturing workflow steps from the virtual design characteristics to the post‐processing methods and the fabrication discrepancy of the printer used (Bártolo 2011, Piedra‐Cascón et al. 2021a, Taormina et al. 2018). Therefore, the characteristics and accuracy of the AM dental device would be significantly impacted by the operator’s choices handling the manufacturing procedures, such as inadequate control over the insufficient thickness of the virtual device design, selection of the different printing parameters, slicing methods, and supportive processes, and the precise execution of the post‐processing techniques (Table 4.1).
Table 4.1 Factors that influence on the accuracy of additively manufactured dental devices.
Manufacturing trinomial | Internal factors | |
---|---|---|
CAD | CAM | |
Additive manufacturing technology Printer
Material |
Geometry of the device Minimum thickness Dental device parameters
|
Printing parameters
Slicing
Post‐processing |

Figure 4.2 Additive manufacturing categories.
The ISO defined accuracy by two terms, namely trueness and precision (ISO 5725‐1). Trueness reports to the ability of a 3D printer to fabricate a CAD object as close to its digital true dimensions, and precision designates the degree of reproducibility of an object by a 3D printer under the same conditions. As 3D objects are produced, the accuracy should be provided on the x‐, y‐, and z‐axes, where the x‐ and y‐axes correspond to the horizontal planes and the z‐axis represents the vertical plane. Furthermore, the accuracy of a printer will be influenced by the printer resolution or the minimum feature size that the printer can reproduce in the x‐ and y‐axes (horizontal) and z‐axis (vertical) resolution.
Different AM categories have been identified independently of the material processed, namely vat‐polymerization, material jetting, material extrusion, powder bed fusion, sheet lamination, and direct energy deposition (Figure 4.2) (ISO:17296‐2:2015). However, in dentistry, not all of the AM categories are normally used to process polymeric, metal, and ceramic dental devices and/or dental prostheses (Javavid and Haleem 2019, Revilla‐León and Özcan 2017, Revilla‐León and Özcan 2019, Revilla‐León et al. 2019a, Revilla‐León et al. 2020a,b, Torabi et al. 2015).
4.3 Polymer Additive Manufacturing
In dentistry, vat‐polymerization, material jetting technologies, and material extrusion have been frequently used to process polymers and fabricate dental devices, such as dental casts, custom trays, silicone indexes, positioning guides for custom abutments, tooth preparation guides, interim dental restorations, removable prostheses, occlusal devices, and surgical guides (Revilla‐León and Özcan 2019, Revilla‐León et al. 2020a) (Table 4.2).
4.3.1 Vat‐Polymerization Technologies
Vat‐polymerization technologies are defined as additively fabricating process in which liquid photopolymer contained in the printer vat is selectively polymerized by a light source (ISO:17296‐2:2015, ISO:52900). Vat‐polymerization technologies can be differentiated into different categories based on the light source used on the 3D printer, namely stereolithography (SLA), direct light processing (DLP), and liquid crystal display (LCD) based printers or daylight polymer printing (DPP) (Javavid and Haleem 2019, Revilla‐León and Özcan 2019, Revilla‐León et al. 2020a). Furthermore, continuous liquid interface production (CLIP) is a DLP modification also considered a vat‐polymerization procedure (Desimone et al. 2014).
Table 4.2 Main dental applications of polymer additive manufacturing technologies.
Polymer printing technologies | Dental applications |
---|---|
Vat‐polymerization
Material jetting |
Diagnostic and definitive casts Surgical guides Endodontic guides Occlusal devices Castable patterns Silicone indexes Custom trays Positioning guides for custom abutments Tooth preparation guides Interim tooth‐ and implant‐supported restorations Removable prostheses Extraoral scan bodies |
Stereolithography technology was conceived by Hideo Kodama of Japan in 1981 (Kodama 1981). In the 1980s, additional patents were obtained by Yoji Marutani of Japan (Marutani 1984), Jean Claude André, Alain Le Méhauté, and Olivier de Witte of France (André et al. 1986), and Charles W. Hull of the United States (Hull 1986). However, the first commercialization of the SLA technology was performed by Professor Hull when he founded 3D Systems (1988). In the SLA technology, a building platform is submerged into a liquid resin, which is polymerized using ultraviolet (UV) light in a layer‐by‐layer manner (ISO:17296‐2:2015, ISO:52900). In SLA technology, a laser traces a cross‐section of the CAD design on the surface of the photosensitive polymer and, subsequently, polymerizes it.
In 1987, Larry Hornbeck from Texas Instruments patented the DLP technology (Hornbeck 2009). While the SLA technology employs a laser to polymerize each layer, in DLP 3D printers, a projector or a digital micromirror device (DMD) projects a mask of light that polymerizes the complete layer of the liquid photopolymer.
The DPP method employs light from LCD screens to polymerize the liquid polymer (Holt 2012). The digital light synthesis (DLS) CLIP procedure selects a DMD to polymerize the resin through an oxygen permeable window composed of fluoropolymer that generates an area named “dead zone” where polymerization is inhibited between the window and the polymerized device (Desimone et al. 2014).
Vat‐polymerization technologies require specific post‐processing procedures after printing the dental device that includes four main steps: removal, cleaning, post‐polymerization, and finishing (Figure 4.3a–d) (Revilla‐León and Özcan 2019). It is recommended to carefully perform the post‐processing methods following the manufacturer’s recommendations for the 3D printer and material selected.
- Removal involves the physical removal of the AM dental object from the building platform. This procedure is carefully performed using a blade, a spatula, or a sharp tool. At this time, the AM device is covered with uncured resin on its surfaces (Revilla‐León and Özcan 2019).
- Cleaning methods remove the unpolymerized liquid polymer from the surface of the AM device by using a bath(s) with an organic solvent such as isopropyl alcohol (IPA), isopropanol alcohol, or tripropylene glycol monomethyl ether (TPM) solvent (Cooperstein et al. 2015, Mostafavi et al. 2021, Sandoval et al. 2007).
- Post‐polymerization is endorsed to perform the complete polymerization of the dental object by using a UV‐polymerization apparatus. The objective of UV post‐polymerization is to improve the degree of conversion and strengthen the layer bonding of the dental device (Gurr et al. 2008, Sandoval and Wicker 2006).43
- Finishing the AM dental device involves the removal of the supporting structures, which can be achieved using a cutting instrument, diamond disk, or ultrasonic tip, depending on the characteristics of the dental polymer selected.
In dentistry, the material and printer manufacturers normally provide the manufacturing guideline or standardized manufacturing protocol, which includes the optimal printing parameters, supportive material, and post‐processing processes for a specific material and printer selection. Nonetheless, certain dental resins can be processed in different vat‐polymerization printers due to the wavelength compatibility between the resin and the printer selected. Therefore, the non‐standardized fabricating protocol is established by user’s proficiency (Piedra‐Cascón et al. 2021a, Scherer et al. 2021).
The selection of the settings among the printing parameters and the execution of the post‐processing methods impact on the printing accuracy include: (Favero et al. 2017, Loflin et al. 2019, Mostafavi et al. 2021, Piedra‐Cascón et al. 2021a, Reymus et al. 2019, Zhang et al. 2019), superficial roughness (Arnold et al. 2019), fit of the AM restorations (Park et al. 2019), and mechanical characteristics of the AM dental devices (Chockalingam et al. 2006). However, additional studies are needed to fully understand the effect of the different manufacturing parameters on the outcome of the AM polymeric dental devices.
4.3.2 Material Jetting Technologies
Material jetting, also called polyjet printing, is an AM process in which droplets of feedstock material are selectively deposited and polymerized by UV light, layer‐by‐layer, until the 3D device is built (ISO:17296‐2:2015, ISO:52900). Material jetting printers deposit the layers of the dental photopolymer resin and typically the support material to partially surround it and enable the manufacturing of different materials with different color and/or properties (Fahad et al. 2013, Ligon et al. 2017). Material jetting 3D printers require less post‐processing procedures where the supportive material is removed by heating or water washout (Stansbury and Idacavage 2016).

Figure 4.3 Post‐processing procedures of a vat‐polymerized diagnostic cast. (a) additively manufactured diagnostic cast being remove from the building platform; (b) rinsing procedures for cleaning the unpolymerized resin located on the surfaces of the additively manufactured diagnostic cast; (c) diagnostic cast after post‐polymerization methods; (d) removing the supportive material from the additively manufactured diagnostic cast.
To maximize the manufacturing accuracy and characteristics of the AM dental devices, it is recommended to follow manufacturing protocol including the optimal printing parameters and post‐processing methods for a particular material and printer choice recommended by the manufacturers.
4.3.3 Material Extrusion
Fused deposition modeling (FDM) technology was developed and patented by Scott Crump who founded the Stratasys Company (Crump 1992). Material extrusion or FDM process is an AM procedure in which a thermoplastic material is selectively dispensed through a nozzle, where it is heated and then deposited in a layer. After the completion of the first layer, the extrusion heads move up or the building platform moves down to facilitate the delivery of the subsequent layer of material. The procedure is repetitive until the 3D object is manufactured (ISO:17296‐2:2015, ISO:52900).
Material extrusion comprises 3D extrusion of thermoplastic thin filament of polymers that are mechanically fed from a spool into the extrusion nozzle (Yardimci et al. 1995). Fused deposition modeling enables the processing of multiple materials, such as rubbers, silicones, organic and inorganic pastes, thermosets, or living cells (Yardimci et al. 1995). However, in dentistry, FDM is not normally selected to manufacture polymeric dental devices due to the lower resolution and finishing surface characteristics.
4.4 Dental Applications of Polymer Additive Manufacturing Technologies
4.4.1 Diagnostic and Definitive Casts
Additively manufactured casts can be obtained using any of the polymer AM technologies reviewed, following manufacturer’s recommendations (Figure 4.4). Manufacturing accuracy depends on the technology, 3D printer, resin material selected, and the manufacturing protocol followed (Etemad‐Shahidi et al. 2020). Additional designing features, such as base model design (Camardella et al. 2017, Rungrojwittayakul et al. 2020, Sherman et al. 2020), model thickness, or the use of a posterior transverse supporting bar (Rungrojwittayakul et al. 2020), which are determined during the virtual design of the dental casts, all influence the accuracy of the AM casts.
Definitive casts for fabricating implant‐supported prostheses can also be fabricated using AM technologies (Figure 4.5a,b). The main difference compared with conventional definitive implant stone casts is the design of the implant analogs. In conventional casts, the implant analogs have a retentive design, so it gets stable and retained into the dental stone; however, in AM casts, the implant analogs are retriable and are positioned into the AM cast after its manufacturing (Figure 4.6a,b) (Revilla‐León et al. 2018a). Furthermore, similar implant analog position accuracy on the AM casts have been reported compared with conventional methods (Banjar et al. 2021, Olea‐Vielba et al. 2020, Papaspyridakos et al. 2020, Revilla‐León et al. 2018a).
The application of the AM casts is a critical factor that might determine the clinically acceptable manufacturing accuracy for fabricating diagnostic and definitive casts using AM technologies (Etemad‐Shahidi et al. 2020). A broad range of a clinically acceptable manufacturing accuracy has been reported varying from 100 to 500 μm for AM diagnostic casts (Brown et al. 2018, Hazeveld et al. 2014, Rungrojwittayakul et al. 2020); however, the acceptable manufacturing accuracy for definitive casts when fabricating tooth‐ and implant‐supported prosthesis remains unclear.
The American Board of Orthodontics allows for the American Board Examination on the usage of AM diagnostic casts using a solid and horseshoe shape base design (Rossini et al. 2016) (Figure 4.7). However, different studies have identified the base design as a factor that can also influence the accuracy of AM diagnostic casts (Revilla‐León et al. 2021c,d). The optimal base design varies depending on the technology, printer, and material selected and the manufacturing protocol used (Revilla‐León et al. 2021c,d).

Figure 4.4 (a) Maxillary and mandibular dentate diagnostic cast; (b) maxillary edentulous diagnostic cast.

Figure 4.5 (a) Additively manufactured definitive cast; (b) detail of surface roughness of additively manufactured definitive cast.

Figure 4.6 (a) Additively manufactured implant definitive cast; (b) detail of surface roughness of additively manufactured definitive implant cast.

Figure 4.7 Additively manufactured cast designed with different base cast designs, namely solid, honeycomb structure, and hollowed designs.
4.4.2 Surgical Implant Guides
Computer‐aided surgical implant planning has reported improved implant placement accuracy compared with free‐hand conventional methods and reduced surgical complications (Chen et al. 2019a, Kernen et al. 2020, Siqueira et al. 2020, Tattan et al. 2020). Surgical implant guides are currently manufactured using either subtractive or AM methods. Furthermore, a dual‐material additively manufactured surgical implant guide has been described (Piedra‐Cascón et al. 2021b). After the manufacturing of the implant surgical guide, a metal sleeve is inserted into the guide (Figure 4.8a,b). Additionally, bone reduction and surgical implant guides can be also designed using implant planning design software programs and manufactured using polymer AM technologies (Figure 4.9a–c).
The tolerance of guide holes in the implant surgical guide is an important factor that can impact the implant placement accuracy; these guide holes are specifically designed for the guide drills of the implant diameter and system selected. Different studies have evaluated the deviation that can occur while drilling due to the tolerance of the drill in the drill key (Koop et al. 2013, Van Assche and Quirynen 2010). Furthermore, the use of a sleeveless implant surgical guide has been described, but limited studies have analyzed its accuracy (Oh et al. 2019).

Figure 4.8 (a) Additively manufactured implant surgical guide before removing the supportive structures; (b) additively manufactured implant surgical guide after post‐processing procedures.

Figure 4.9 (a) Additively manufactured positioning guide before extraction procedures; (b) additively manufactured guide for bone reduction procedure before implant placement; (c) additively manufactured implant surgical guide.
The accuracy of both milled and AM implant surgical guides have been analyzed in the dental literature (Abduo and Lau 2020, Henprasert et al. 2020), but contradictory results can be found. Previous studies have reported higher (Abduo and Lau 2020) or similar (Henprasert et al. 2020) accuracy while using additively manufactured compared with milled surgical ones.
Similarly, as in the previous dental applications of AM polymer technologies, the accuracy of surgical implant guides will depend on the operator’s decision regarding the printer, technology, and polymer chosen and the determination of the printing parameters and post‐processing methods performed (Chen et al. 2019b, Gjelvold et al. 2019, Herschdorfer et al. 2021, Ozan et al. 2009, Rubayo et al. 2021, Turbush and Turkyilmaz 2012, Yeung et al. 2020).
Once the AM surgical implant guide is manufactured, it can be sterilized following the manufacturer’s recommendations to ensure that the implant surgical guide does not suffer volumetric changes (Marei et al. 2019, Sharma et al. 2020, Török et al. 2020).
4.4.3 Endodontic Guides
Cone beam computed tomography images are aligned with intraoral digital scans or digitized diagnostic casts and used to design the endodontic guide. Additively manufactured guides have been developed for endodontic treatments, such as conservative access, finding calcified, or missing canals, and accomplishing precise osteotomy in apicoectomies (Connert et al. 2019, Kostunov et al. 2021, Llaquet Pujol et al. 2021, Loureiro et al. 2020, Torres et al. 2021, Sato et al. 2021). Furthermore, guided endodontics provides faster access with higher predictability and more conservative preparation compared with conventional methods (Connert et al. 2019, Loureiro et al. 2020).
4.4.4 Occlusal Devices
Polymer AM technologies have also been selected to manufacture a single material (Lauren and McIntyre 2008, Waldecker et al. 2019) and dual‐material occlusal devices (Piedra‐Cascón et al. 2020) using vat‐polymerization AM technologies.
Similar to any dental device manufactured using AM technologies, the outcome of the AM occlusal device varies based on user choices with regard to technology, printer, and material selected, together with the printing parameters and post‐processing procedures performed. Furthermore, the virtual design is a fundamental element on the quality of the AM occlusal device that should be done carefully by the dental professional. The designing procedures, normally performed using a CAD software program, require the control of diverse design variables, such as device path of insertion, undercut area location and posterior block‐out, device extensions, minimum material thickness, and tooth offset (Figure 4.10a,b). Although CAD software programs provide recommended settings for designs of specific devices, dental professionals would normally determine the characteristic design based on their expertise.
Studies that assessed the mechanical properties of dental resins for the fabrication of occlusal devices are sparse but have described that AM materials for occlusal devices have comparable properties to those of conventional materials (Berntsen et al. 2018, Huettig et al. 2017, Salmi et al. 2013, Väyrynen et al. 2016). A previous study has compared the fit of occlusal appliances made of rigid material with a layer thickness of 100 μm and manufactured by using a vat‐polymerization printer with three build orientations (0°, 30°, and 90 ). The authors concluded that the best build orientation to ensure proper fit was 0° (Vasques and Laganá 2018). Salmi et al. described a workflow to manufacture an occlusal device by using a vat‐polymerization printer with a rigid material. Patients receiving the AM occlusal device reported it to be excessively tight at every use (Salmi et al. 2013). Further studies are recommended to determine the optimal parameters of the AM occlusal devices, which are dependent on the manufacturing trinomial, namely technology, printer, and resin used.
4.4.5 Castable Patterns
Additively manufactured castable patterns can be used for the fabrication of casted metal or pressed ceramic prosthesis (Dickens 1993). Williams et al. reported the first clinical documentation of an AM pattern for the fabrication of the casted metal framework of a removable partial denture (RPD). The technique described included the digitalization of the definitive casts using a laboratory scanner, which allowed the obtention of the virtual working casts. The digital design comprised the determination of the path of insertion, surveying the cast, location of the undercuts, and virtual design of the elements of the metal framework, such as major and minor connectors, proximal plates, occlusal rests, or clasps (Eggbeer et al. 2005, Williams et al. 2004, Williams et al. 2006).
The accuracy of the metal frameworks fabricated using AM castable methods have been analyzed in the dental literature reporting significant differences on the manufacturing accuracy depending on the fabricating technique and location/area of the metal framework (Lee et al. 2017b, Tasaka et al. 2020, Tasaka et al. 2021) but seem to provide a clinically acceptable technique.
Additively manufactured castable patterns have been described to manufacture casted titanium (Wu et al. 2001) and pressed lithium disilicate ceramic restorations, such as inlays, onlays, tooth‐supported and cement‐retained implant‐supported crowns (Homsy et al. 2018a,b, Revilla‐León et al. 2018b, Taha et al. 2018). However, studies provide inconclusive data for the marginal and internal discrepancies of the ceramic restorations manufactured from AM castable patterns compared with milled or handmade patterns (Homsy et al. 2018a,b, Revilla‐León et al. 2018b, Taha et al. 2018).

Figure 4.10 Additively manufactured occlusal device: (a) additively manufactured occlusal device; (b) maxillary occlusal view without the occlusal guard; (c) occlusal guard placed on the maxillary arch.
4.4.6 Silicone Indices
A silicone index is normally used to translate the diagnostic waxing information to the patient’s mouth for the fabrication of diagnostic trial restorations, direct composite restorations, fiber‐reinforced FDPs, interim restorations, or guide a tooth preparation. Conventionally, the silicone index is fabricated in one‐piece using the diagnostic waxing stone cast. Digital technologies provide different diagnostic and manufacturing tools for the virtual design and AM of the one‐piece index (Revilla‐León et al. 2018c).
The development of AM applications in dentistry have facilitated the broad range of materials available, including clear rigid and clear flexible polymers that combined with the CAD software program design capabilities have allowed the manufacturing of dual‐material one‐ (Revilla‐León et al. 2019b,c), three‐ (Gonzalez et al. 2020, Park et al. 2020b) and four‐piece AM silicone indexes (Revilla‐León et al. 2020d) (Figure 4.11).
Nonetheless, the surface characteristics of the AM index obtained during the layer‐by‐layer build up can be translated to the diagnostic trial restorations, direct composite restorations, fiber‐reinforced FDPs, and interim restorations. A previous study has assessed the influence of different print angulations (0°, 25°, 45°, 75°, and 90°) on the surface roughness of the intaglio of AM indices (Revilla‐León et al. 2020e). The results of this study suggested to print the index at 0° print orientation to minimize the surface roughness on the intaglio of the index and, as a result, a smoother finished surface on the restoration.
4.4.7 Custom Trays
Vat‐polymerization, material jetting, or material extrusion methods can be chosen to fabricate custom trays for conventional impression procedures. Digital technologies facilitate the uniform impression material space while designing the custom tray (Piedra‐Cascón and Revilla‐León 2018, Revilla‐León et al. 2017) and baseplate and occlusion rims (Piedra‐Cascón et al. 2019) (Figure 4.12a,b).
It is important to remember that the manufacturing accuracy of a custom tray is not as critical as for other dental devices such as interim dental restorations (Liu et al. 2021). One study evaluated the adhesion between the impression materials and custom trays manufactured using material extrusion technology (Xu et al. 2018). The results showed that the surface roughness of the AM custom trays benefited the adhesion between the polymer tray and the impression materials, and therefore, post‐processing polishing methods on AM custom trays are not recommended.

Figure 4.11 Additively manufactured three‐piece silicone index.
Previous authors have described the design using a CAD software program and vat‐polymerizing of a custom tray combined with an intraoral gothic arch in a completely edentulous patient (Qu et al. 2019). The AM custom trays eased the conventional impression procedures, and the gothic arch enabled the interocclusal registration in the same clinical appointment. Furthermore, the removable design of the mandibular gothic arch plate facilitated the tongue movement while performing the conventional impression of the edentulous mandibular arch.
4.4.8 Interim Dental Restorations
Manufacturing of tooth‐ and implant‐supported interim dental prostheses have been broadly reported in the dental literature (Revilla‐León and Özcan 2019), (Figure 4.13a,b); however, the chemical composition, biocompatibility, mechanical properties, and clinical behavior are still under investigation, which can justify its clinical use (Revilla‐León et al. 2019a).
Additively manufactured interim dental materials present major chemical composition variations compared with conventional materials (Revilla‐León et al. 2020c). However, a previous study showed that AM interim dental materials obtained superior cytocompatibility, better cell adhesion, and higher cell proliferation compared with that in conventional resin materials (Park et al. 2020c).
It is important to remember that the manufacturing trinomial, printing parameters, slicing method, support structures, and post‐processing techniques influence the manufacturing accuracy and characteristics of AM interim dental restorations (Alharbi et al. 2016a,b, Osman et al. 2017a, Ryu et al. 2020). The generalization of the optimal manufacturing procedure is very complex, as it varies depending on the technology, printer, and material selected. Furthermore, comparisons among the different studies published is not possible due to variations of the manufacturing methods of the AM specimens and the measurement techniques employed.

Figure 4.12 (a) Additively manufactured custom tray for a complete‐arch implant impression procedure; (b) detail of the surface roughness of the additively manufactured custom tray

Figure 4.13 (a) Additively manufactured interim dental crown; (b) additively manufactured interim shell for the elaboration of a tooth‐supported interim prostheses.
An essential consideration is the layer orientation related to the load direction that the interim dental restoration will receive. When the layer of the device is perpendicular to the load direction, the compressive strength is higher than when the layer is oriented parallel (Alharbi et al. 2016a).
The internal and marginal fit of AM interim crowns have been analyzed in the literature (Chaturvedi et al. 2020, Lee et al. 2017a, Mai et al. 2017). The majority of the studies reported better internal and marginal fit when manufacturing the restorations using AM methods compared with subtractive or conventional techniques (Bae et al. 2020, Chaturvedi et al. 2020, Lee et al. 2017a, Mai et al. 2017).
Furthermore, AM interim crowns seem to provide adequate mechanical properties (Park et al. 2020a, Reymus et al. 2020), including the repairing capabilities of those printing interim restorations using composite resin material (Albahri et al. 2021, Revilla‐León et al. 2020c) compared with current subtractive and conventional interim restorations. However, artificial aging studies suggested that aging affects the mechanical properties of AM interim materials, and, therefore, are not recommended to use as long‐term interim restorations (Nold et al. 2021, Reymus et al. 2020).
A new technique to fabricate additively manufactured ingot for milling interim dental restorations has been described (Pérez‐Giugovaz et al. 2021b). This method consists of additively manufacturing a one‐piece ingot using a vat‐polymerization printer, which is used to mill the interim dental restoration. This technique aims to minimize the manufacturing time while also reducing the cost and the reduction of the surface roughness of the interim dental restoration.
4.4.9 Removable Prostheses
Complete and partial removable prostheses can also be fabricated using 3D polymer printers (Figure 4.14a–d). The majority of the studies employed vat‐polymerization and material jetting methods, due to the high manufacturing accuracy compared with material extrusion techniques (Anadioti et al. 2020). In the first published report in English, dated 1994, the authors employed an SLA 3D printer from digitized conventional impressions (Maeda et al. 1994).
Additive manufacturing of complete dentures normally involves fabricating the denture base and the denture teeth independently using a polymer 3D printing. Afterward, both printed parts are bonded together using a light‐polymerization bonding agent (Choi et al. 2020, Choi et al. 2021, Chung et al. 2018). Additionally, commercially available denture teeth can also be used and bonded to additively manufactured denture bases, and, to do so, during the digital design of the complete dentures, the commercially available teeth should be selected on the denture teeth library of the CAD software program.
Limited studies in the dental literature have analyzed and compared the fracture resistance (Chung et al. 2018) or wear resistance (Cha et al. 2020) of AM and commercially available denture teeth. Results suggest that AM denture teeth might provide adequate properties for their clinical use (Cha et al. 2020, Chung et al. 2018).
The manufacturing accuracy of AM complete dentures is affected by the manufacturing trinomial, printing parameters, and post‐processing methods performed (Tasaka et al. 2019). The generalization of the optimal printing parameters for a specific printer, technology, and denture material is still under development (Gao et al. 2021, Hada et al. 2020, Hsu et al. 2020, Inokoshi et al. 2012, Jin et al. 2020, Kalberer et al. 2019, Kraemer Fernandez et al. 2020, Masri et al. 2020).

Figure 4.14 (a) Additively manufactured maxillary denture trial; (b) additively manufactured maxillary and mandibular complete denture; (c) implant‐supported maxillary denture trial; (d) detail of the surface roughness of the AM denture teeth trial.
Different studies have compared milled and additively manufactured complete dentures, reporting improved manufacturing accuracy and similar or better intaglio adaptation when manufacturing with AM techniques; however, milled prostheses obtained better polishability (Hsu et al. 2020, Hwang et al. 2019, Wang et al. 2021, Yoon et al. 2018, Yoon et al. 2020).
A retrospective clinical study aimed to compare the post‐insertion maintenance visits between conventional and AM complete dentures (Kim et al. 2021). The AM complete denture group obtained a significantly lower pain and visible ulcer lesions on the oral mucosa, patient’s discomfort, and esthetic satisfaction; however, similar clinical performance was reported for both manufacturing method groups.
A clinically efficient method described in the literature is the duplication of existing dentures (Schmidt et al. 2020, Takeda et al. 2020, Yang et al. 2021) or printed denture flask (Kim et al. 2020) using 3D polymer printers. The AM duplicated complete dentures can be used as custom trays and/or denture trials during the elaboration of new complete dentures, implant‐supported and implant‐retained prostheses (Kim et al. 2020, Lo Russo et al. 2020, Schmidt et al. 2020, Takeda et al. 2020, Yang et al. 2021).
4.4.10 Extraoral Scan Bodies for Virtual Patient Integration
Polymer AM processes can be also used to manufacture extraoral scan bodies (Figure 4.15a,b) that are used to guide the alignment procedures between facial and intraoral digital scans and obtain a virtual 3D patient’s representation (Pérez‐Giugovaz et al. 2021c, Pérez‐Guigovaz et al. 2022). The alignment protocol consists of acquiring intraoral digital scans using an intraoral scanner, a facial scan with the intraoral scan body stabilized in the patient’s mouth using polyvinyl siloxane impression dental material, and, after removing the intraoral scanner, different facial scans with the patient at different positions such as rest or smile. Thereafter, the AM intraoral scan body is digitized using a laboratory scanner following the scanning protocol endorsed by the manufacturer. Subsequently, all of the patient’s digital information is merged, and a virtual patient visualization is attained (Pérez‐Giugovaz et al. 2021a). This information can be also transferred to the virtual articulator as described by Pérez‐Giugovaz et al. (2021c).

Figure 4.15 (a) Additively manufactured scan body with hollow occlusal surface design (Sat3D; Sat3D); (b) Additively manufactured scan body with solid occlusal surface design (Sat3D; Sat3D).
As an advantage, the virtual design of the intraoral scan body can be customized to adapt to a patient’s mouth dimensions to improve patient’s comfort while scanning and being fabricated in the office using a chairside 3D printer (Pérez‐Giugovaz et al. 2021a).
Another interesting application is the fabrication of additively manufactured intraoral scan bodies to facilitate complete‐arch implant digital scans (Pérez‐Giugovaz et al. 2022). Similarly, the intraoral scan body can be customized to the patient’s mouth dimensions enhancing the patient’s comfort.
4.5 Metal Additive Manufacturing
Subtractive and additive techniques have been defined as hardware or software dependent, where performance is dependent on the ability of a virtual design compressed in a CAD file, typically in an STL file format, to be manufactured as a 3D device (Koutsoukis et al. 2015). Nonetheless, the dental literature comparing the manufacturing accuracy of metal devices using additive and subtractive methods is scarce, but limited dental investigations have described comparable manufacturing accuracy and none of the manufacturing procedures tested were able to fabricate a perfect match to the virtual CAD design (Bae et al. 2017, Braian et al. 2018).
Powder bed fusion technologies are the most frequent metal AM technology used to manufacture cobalt–chromium (Co–Cr) and titanium (Ti) frameworks in restorative dentistry (Revilla‐León and Özcan 2017, Revilla‐León et al. 2020f). There are three main categories of powder bed fusion technologies, namely selective laser sintering (SLS), selective laser melting (SLM), and electron beam melting (EBM). The main concept consists of using the powder of a metal alloy, which will be sintered or melted to build up a metal framework by adopting a layer‐by‐layer method.
After manufacturing a metal framework using PBF technology, the residual powder that is non‐melted might be recycled or wasted (Jacob et al. 2017). Nonetheless, there is no specific protocol for the recycling processes and there is limited literature regarding how the grain features of a recycled powder would impact the mechanical properties of the AM framework (O’Leary et al. 2015, Seyda et al. 2012, Tang et al. 2015).
Similarly, as polymer AM technologies, the manufacturing workflow includes data processing, manufacturing, and post‐processing. The AM metal also requires post‐processing methods with the purpose of surface modification to correct morphology and roughness. The post‐processing techniques can include mechanical abrasion, electro‐polishing, sandblasting, computer numerical control (CNC) machining, chemical mechanical polishing, and laser polishing. In dentistry, the most common post‐processing procedures are sandblasting and CNC machining. Additive manufacturing frameworks for RPDs or tooth‐supported prostheses are treated with air‐particle abrasion, while AM frameworks for implant‐supported prostheses are also treated by subtractive technologies after printing the framework; the implant interface is milled.
4.5.1 Selective Laser Sintering
In 1989, Carl Deckard and Joe Beaman developed the SLS technology (Deckard 1986). Selective laser sintering contains a high‐powered laser (Nd:YAG laser) beam that selectively melts metal powder into a solid layer (20–100 μm). Subsequently, another layer of metal powder is disposed, and the next slice of the metal device is attached with the first. This process reiterates until the framework is fabricated. The manufacturing temperature does not reach the melting point of the selected metal; hence, the metal powder sinters and does not completely melt (Fisher et al. 2002).
4.5.2 Selective Laser Melting
Selective laser melting printers use high‐quality lasers (CO2 or Nd:YAG laser) that facilitate the complete melting of the metal powder in an inert chamber with purified argon or nitrogen (Murr et al. 2009, Traini et al. 2008). The building platform is heated to a temperature typically up to 200°C (Tan et al. 2017). The particle size of the powder ranges between 20 and 60 μm (Tan et al. 2017). The SLM procedure accumulates high internal stresses due to manufacturing thermal changes and a post‐processing heat treatment has been endorsed (Kruth and Mercelis 2006).
4.5.3 Electron Beam Melting
Electron beam melting printers employ an electron beam to selectively melt metal powder layers in an inert vacuum chamber with purified argon (Larson 1993). During the manufacturing process, a high temperature (approx. 700°C) is sustained in the chamber of the metal printer to reduce temperature gradients and diminish residual stresses. First, a tungsten filament is heated to over 3000°C, triggering electrons to be emitted and accelerated due to the potential difference between an anode and a cathode. The electrons are focused using magnetic coils to form a narrow high‐energy beam that strikes the surface of the metal powder. At this point, the kinetic energy transferred through friction creates the necessary heat to melt the metal powder (Murr et al. 2012). The metal powder’s grain size usually ranges from 45 to 150 μm (Triantaphyllou et al. 2015, Wang et al. 2017).
The application of metal AM technologies in dentistry is growing and the implementation in different clinical dental interventions is increasing. However, limited dental literature has assessed and compared the chemical composition, microstructure (Al Jabbari et al. 2014, Kim et al. 2016, Koutsoukis et al. 2015, Qian et al. 2015, Takaichi et al. 2013), and mechanical properties (Bilgin et al. 2016, Choi et al. 2014, Han et al. 2018, Niinomi 2008, Zhou et al. 2018) of metal dental devices manufactured using subtractive and additive methods.
Overall, variations in chemical compositions among the dental alloys manufactured using different techniques can be expected (Revilla‐León and Özcan 2017, Revilla‐León et al. 2020f). Furthermore, studies have demonstrated a strong correlation between the manufacturing procedure and the metal microstructure, where casted metal specimens presented high internal porosity, milled and techniques obtained by AM offered dense specimens without internal porosity (Al Jabbari et al. 2014, Koutsoukis et al. 2015, Wu et al. 2014). Additionally, previous investigations revealed similar or better mechanical properties (Al Jabbari et al. 2014, Koutsoukis et al. 2015, Kim et al. 2016) and similar ceramic bond strength compared with milled and casted dental alloys (Barazanchi et al. 2020, Li et al. 2017, Serra‐Prat et al. 2014, Wu et al. 2014).
4.6 Dental Applications of Metal Additive Manufacturing Technologies
Powder bed fusion AM technologies are typically used in restorative dentistry to manufacture Co–Cr frameworks for RPDs and complete dentures, Co–Cr frameworks for tooth‐supported metal‐ceramic crowns and FDPs, Co–Cr and Ti frameworks for implant‐supported prostheses, and Co–Cr frameworks for implant impression techniques (Revilla‐León and Özcan 2017, Revilla‐León et al. 2020f, Revilla‐León et al. 2021b); (Table 4.3).
4.6.1 Metal Frameworks for Removable Partial Dentures
Specific dental CAD software programs facilitate the virtual design of RPDs, including the metal framework design. Typically, the design is initiated following the digitalization of definitive working casts. The CAD software program allows the path of insertion selection, undercuts location, and design of all RPD framework components. As a result, the virtual design of the metal framework, in a STL file format, is exported and used to additively manufacture the metal framework of the dental prostheses.
Table 4.3 Main dental applications of metal additive manufacturing technologies.
Metal printing technologies | Dental applications |
---|---|
Powder bed fusion technologies
|
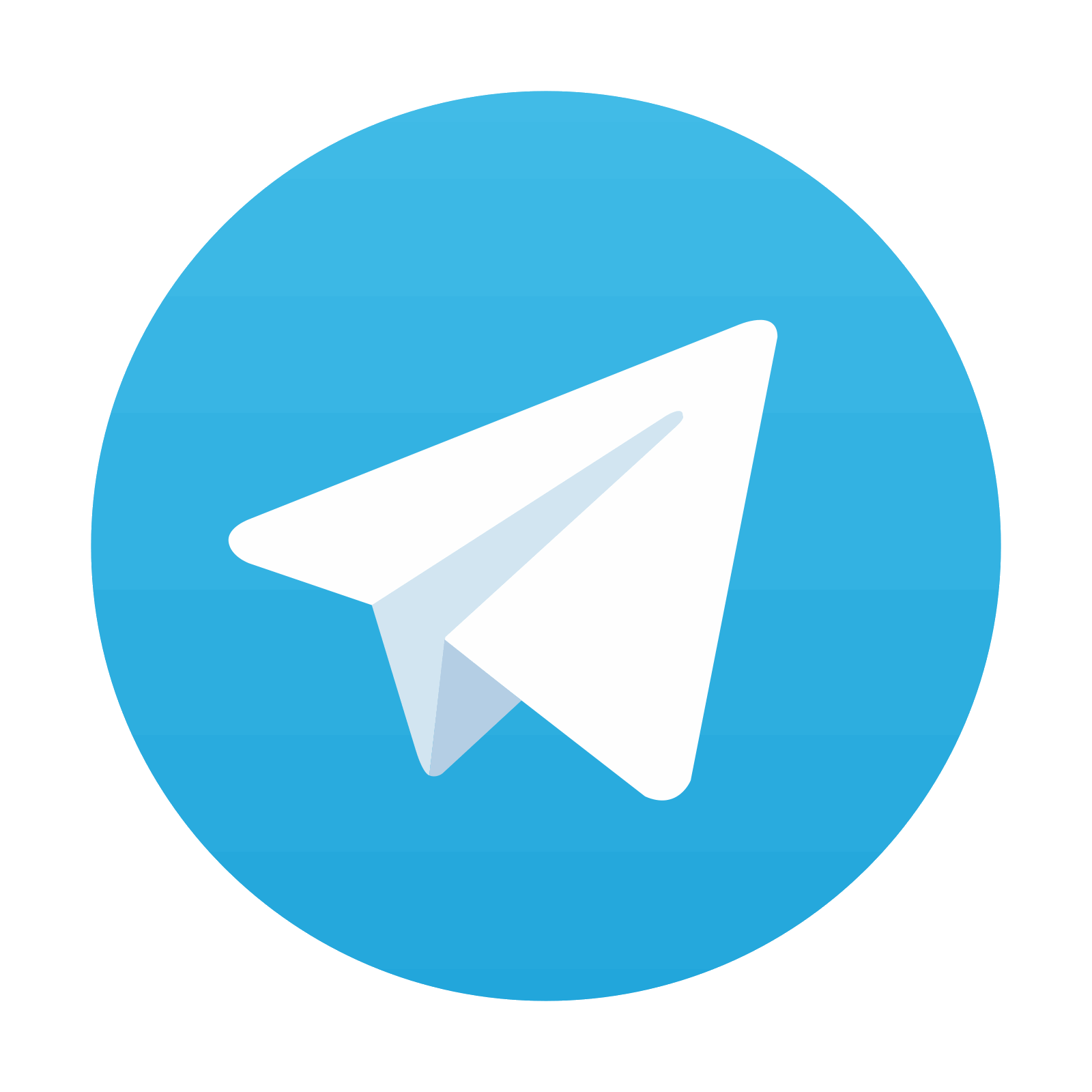
Stay updated, free dental videos. Join our Telegram channel

VIDEdental - Online dental courses
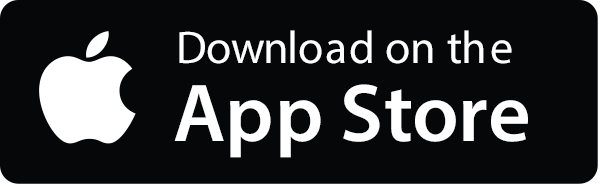
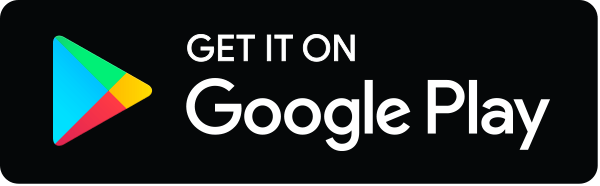