Physics
One atom says to a friend, “I think I lost an electron.” The friend replies, “Are you sure?” “Yes,” says the first atom, “I’m positive.”
Dentists make radiographic images of patients when they seek additional information beyond that available from a clinical examination or their patient’s history. Dentists combine the information from these images with their findings from the clinical examination and history to form a diagnosis. When a diagnosis is established, treatment can be provided. This chapter considers the initial steps in making radiographic images, including the operation of an x-ray machine and the interactions of radiation with matter.
Composition of Matter
Matter is anything that has mass and occupies space. All visible matter in the universe (all stable matter) is made of up quarks, down quarks, and electrons. These particles are fundamental because they have no inner structure and cannot be divided. Up quarks and down quarks combine to form neutrons and protons in atomic nuclei. Electrons are located in orbitals outside the nuclei. Historically, the atom has been viewed as a miniature solar system with a nucleus at the center and revolving electrons. This classic view of the atom has been replaced by the Standard Model, which describes the subatomic particles (Table 1-1), and the Quantum Mechanical Model, which describes the arrangement of electrons in an atom. In addition to matter particles, the Standard Model also describes force carrier particles—particles that mediate interactions between matter particles (Table 1-2).
TABLE 1-1
FAMILIES OF PARTICLES | ||||||||||
Charge | I | II | III | |||||||
Quarks | +![]() |
Up | u | Charm | c | Top | t | |||
−![]() |
Down | d | Strange | s | Bottom | b | ||||
Leptons | −1 | Electron | e | Muon | µ | Tau | τ | |||
0 | Electron neutrino | νe | Muon neutrino | νµ | Tau neutrino | ντ |
TABLE 1-2
Particle | Symbol | Action |
Photon | γ | Make up x-ray beams and mediate electromagnetic interactions |
Gluon | g | Mediate strong nuclear force that binds quarks into protons and neutrons and bind nuclei together |
W boson | W | Mediate weak interactions; associated with beta decay |
Z boson | Z | Mediate weak interactions; associated with neutrino scatter |
Atomic Structure
Nucleus
In all atoms except hydrogen, the nucleus consists of positively charged protons and neutral neutrons. A hydrogen nucleus contains a single proton. Protons and neutrons are made of quarks (Fig. 1-1). Protons consist of two up quarks and one down quark and thus have a charge of +1. Neutrons are made of one up quark and two down quarks and thus are neutral. Although the positively charged protons repel each other, the nucleus is held together by the strong nuclear force, the rapid exchange of gluons. The strong nuclear force overwhelms the repulsive electromagnetic effect at the incredibly short distances inside an atomic nucleus.
The number of protons in the nucleus determines the identity of an element. This is its atomic number (Z), the nuclear charge. A change in the number of protons in an atom changes it to another element. Each of the more than 100 elements has a unique atomic number, a corresponding number of orbital electrons in the ground state, and unique chemical and physical properties. The total number of protons and neutrons in the nucleus of an atom is its atomic mass (A). A change in the number of neutrons in an atom changes the stability of the element. Nearly the entire mass of the atom consists of the protons and neutrons in the nucleus.
Electron Orbitals
Electrons exhibit both particle-like properties (e.g., they have mass) and wavelike properties (e.g., they generate interference patterns). Electrons exist within three-dimensional volumes called orbitals. Orbitals represent the probability locations of the electron in space at any instant in time—the regions in which the electron is most likely to exist. The letters s, p, d, f, g, and h are used to describe orbital shapes (Fig. 1-2). These letters replace the K, L, M, N, O, and P designations previously used. Only two electrons may occupy an orbital. The s-type orbital is spherical. The s-type orbitals are the first to be filled in every element. Next are the p-type orbitals, which are bilobed and centered on the nucleus. Next are the d-type orbitals, which consist of four lobes arranged around the nucleus—they are bilobed with a ring. In an atom with many electrons, the electron clouds of one orbital are superimposed with the electron clouds of other orbitals. No known atom has more than seven orbitals. Electrons occupy the lowest energy available orbitals—those not already occupied by other electrons. A change in the number of electrons of an atom changes the charge of an atom.
Ionization
When the number of electrons in an atom is equal to the number of protons in its nucleus, the atom is electrically neutral. If a neutral atom loses an electron, it becomes a positive ion, and the free electron becomes a negative ion. This process of forming an ion pair is termed ionization. Ionizing an atom requires sufficient energy to overcome the electron binding energy, the electrostatic force binding the electrons to the nucleus. The binding energy of an electron is related to the atomic number of the atom and the orbital type. Elements with a large atomic number (high Z) have more protons in their nucleus and thus bind electrons in any given orbital more tightly than smaller Z elements. Within a given atom, electrons in the inner orbitals are more tightly bound than the more distant outer orbitals. Tightly bound electrons require the energy of x rays or high-energy particles to remove them, whereas loosely bound outer electrons can be displaced by ultraviolet radiation. However, nonionizing types of radiation, such as visible light, infrared, and microwave radiation, and radio waves do not have sufficient energy to remove bound electrons from their orbitals.
Nature of Radiation
Radiation is the transmission of energy through space and matter. It may occur in two forms: (1) particulate (Table 1-3) and (2) electromagnetic. Natural radioactivity and radiation therapy may involve both particulate and electromagnetic radiation. Oral and maxillofacial radiology involves only electromagnetic radiation.
TABLE 1-3
Particle | Symbol | Elementary Charge* | Rest Mass (amu) |
Alpha | α | +2 | 4.00154 |
Beta+ (positron) | β+ | +1 | 0.000549 |
Beta− (electron) | β− | −1 | 0.000549 |
Electron | e− | −1 | 0.000549 |
Neutron | n0 | 0 | 1.008665 |
Proton | p | +1 | 1.007276 |
amu, Atomic mass units, where 1 amu = the mass of a neutral carbon-12 atom.
*Elementary charge of 1 equals that the charge of a proton or the opposite of an electron.
Particulate Radiation
Small atoms have roughly equal numbers of protons and neutrons, whereas larger atoms tend to have more neutrons than protons. Larger atoms are unstable because of the unequal distribution of protons and neutrons, and they may break up, releasing α (alpha) or β (beta) particles or γ (gamma) rays. This process is called radioactivity. When a radioactive atom releases an α or a β particle, the atom is transmuted into another element. α particles are helium nuclei consisting of two protons and two neutrons. They result from the radioactive decay of many large atomic number elements. Because of their double positive charge and heavy mass, α particles densely ionize matter through which they pass. They quickly give up their energy and penetrate only a few micrometers of body tissue. (An ordinary sheet of paper absorbs them.) After stopping, α particles acquire two electrons and become neutral helium atoms.
An unstable atom with an excess of neutrons may decay by converting a neutron into a proton, a β− particle, and a neutrino. β− particles are identical to electrons. High-speed β− particles are not densely ionizing; they are able to penetrate matter to a greater depth than α particles can—up to 1.5 cm in tissue. This deeper penetration occurs because β− particles are smaller and lighter and carry a single negative charge; they have a much lower probability of interacting with matter than α particles. β− particles from radioactive iodine-131 are used for treatment of some thyroid cancers. An unstable atom with an excess of protons may decay by converting a proton into a neutron, a β+ particle (positron), and a neutrino. Positrons quickly annihilate with electrons to form two γ rays. This reaction is the basis for positron emission tomography scanning (see Chapter 14).
The capacity of particulate radiation to ionize atoms depends on its mass, velocity, and charge. The rate of loss of energy from a particle as it moves along its track through matter (tissue) is its linear energy transfer (LET). A particle loses kinetic energy each time it ionizes adjacent matter. The greater the physical size of the particle, the higher its charge, and the lower its velocity, the greater its LET. For example, α particles, with their high mass compared with an electron, high charge, and low velocity, are densely ionizing, lose their kinetic energy rapidly, and have a high LET. β− particles are much less densely ionizing because of their lighter mass and lower charge; they have a lower LET. High LET radiations concentrate their ionization along a short path, whereas low LET radiations produce ion pairs much more sparsely over a longer path length.
Another type of radioactivity is γ decay. γ rays are photons, a form of electromagnetic radiation (see next section). They result as part of a decay chain where a nucleus converts from an excited state to a lower level ground state; this often happens after a nucleus emits an α or β particle or after nuclear fission or fusion.
Electromagnetic Radiation
Electromagnetic radiation is the movement of energy through space as a combination of electric and magnetic fields. It is generated when the velocity of an electrically charged particle is altered. γ rays, x rays, ultraviolet rays, visible light, infrared radiation (heat), microwaves, and radio waves all are examples of electromagnetic radiation (Fig. 1-3). γ rays originate in the nuclei of radioactive atoms. They typically have greater energy than x rays. In contrast, x rays are produced outside the nucleus and result from the interaction of electrons with large atomic nuclei in x-ray machines. The higher energy types of radiation in the electromagnetic spectrum—ultraviolet rays, x rays, and γ rays—are capable of ionizing matter. Some properties of electromagnetic radiation are best explained by quantum theory, whereas others are most successfully described by wave theory.
Quantum theory considers electromagnetic radiation as small discrete bundles of energy called photons. Each photon travels at the speed of light and contains a specific amount of energy. The unit of photon energy is the electron volt (eV), the amount of energy acquired by one electron accelerating through a potential difference of one volt. The relationship between wavelength and photon energy is as follows:
< ?xml:namespace prefix = "mml" ns = "http://www.w3.org/1998/Math/MathML" />

where E is energy in kiloelectron volts (keV), h is Planck’s constant (6.626 × 10−34 joule-seconds or 4.13 × 10−15 eV-seconds), c is the velocity of light, and λ is wavelength in nanometers. This expression may be simplified to:

Quantum theory has been successful in correlating experimental data on the interaction of radiation with atoms, the photoelectric effect, and the production of x rays. The wave theory of electromagnetic radiation maintains that radiation is propagated in the form of waves, similar to the waves resulting from a disturbance in water. Such waves consist of electric and magnetic fields oriented in planes at right angles to one another that oscillate perpendicular to the direction of motion (Fig. 1-4). All electromagnetic waves travel at the velocity of light (c = 3.0 × 108 m/s) in a vacuum. Waves of all kinds exhibit the properties of wavelength (λ) and frequency (ν) and are related as follows:

where λ is in meters and ν is in cycles per second (hertz). Wave theory is more useful for considering radiation in bulk when millions of quanta are being examined, as in experiments dealing with refraction, reflection, diffraction, interference, and polarization.
High-energy photons such as x rays and γ rays are typically characterized by their energy (electron volts), medium-energy photons (e.g., visible light and ultraviolet waves) are typically characterized by their wavelength (nanometers), and low-energy photons (e.g., AM and FM radio waves) are typically characterized by their frequency (KHz and MHz).
X-Ray Machine
X-ray machines produce x rays that pass through a patient’s tissues and strike a digital receptor or film to make a radiographic image. The primary components of an x-ray machine are the x-ray tube and its power supply. The x-ray tube is positioned within the tube head, along with some components of the power supply (Fig. 1-5). An electrical insulating material, usually oil, surrounds the tube and transformers. Often, the tube is recessed within the tube head to improve the quality of the radiographic image (see Chapter 6). The tube head is typically supported by an arm that is usually mounted on a wall. A control panel allows the operator to adjust the duration of the exposure, and often the energy and exposure rate, of the x-ray beam.
X-Ray Tube
An x-ray tube is composed of a cathode and an anode situated within an evacuated glass envelope or tube (Fig. 1-6). Electrons stream from the filament in the cathode to the target in the anode, where the energy from some of the electrons is converted into x rays. For the x-ray tube to function, a power supply is necessary to:
Cathode
The cathode (Fig. 1-7, B; see also Fig. 1-6) in an x-ray tube consists of a filament and a focusing cup. The filament is the source of electrons within the x-ray tube. It is a coil of tungsten wire about 2 mm in diameter and 1 cm or less in length. Filaments typically contain about 1% thorium, which greatly increases the release of electrons from the heated wire. The filament is mounted between two stiff support wires that carry an electrical current. These two mounting wires lead through the glass envelope and connect to both the high-voltage and the low-voltage electrical sources. The filament is heated to incandescence by the flow of current from the low-voltage source and emits electrons at a rate proportional to the temperature of the filament.
The filament lies in a focusing cup (Fig. 1-7, B; see also Fig. 1-6), a negatively charged concave reflector made of molybdenum. The parabolic shape of the focusing cup electrostatically focuses the electrons emitted by the filament into a narrow beam directed at a small rectangular area on the anode called the focal spot (Fig. 1-7, C, and Fig. 1-8). The electrons move to the focal spot because they are both repelled by the negatively charged cathode and attracted to the positively charged anode. The x-ray tube is evacuated to prevent collision of the fast-moving electrons with gas molecules, which would significantly reduce their speed. The vacuum also prevents oxidation, or “burnout,” of the filament.
Anode
The anode in an x-ray tube consists of a tungsten target embedded in a copper stem (see Figs. 1-6 and 1-7, C). The purpose of the target in an x-ray tube is to convert the kinetic energy of the colliding electrons into x-ray photons. The target is made of tungsten, an element that has several characteristics of an ideal target material, including the following:
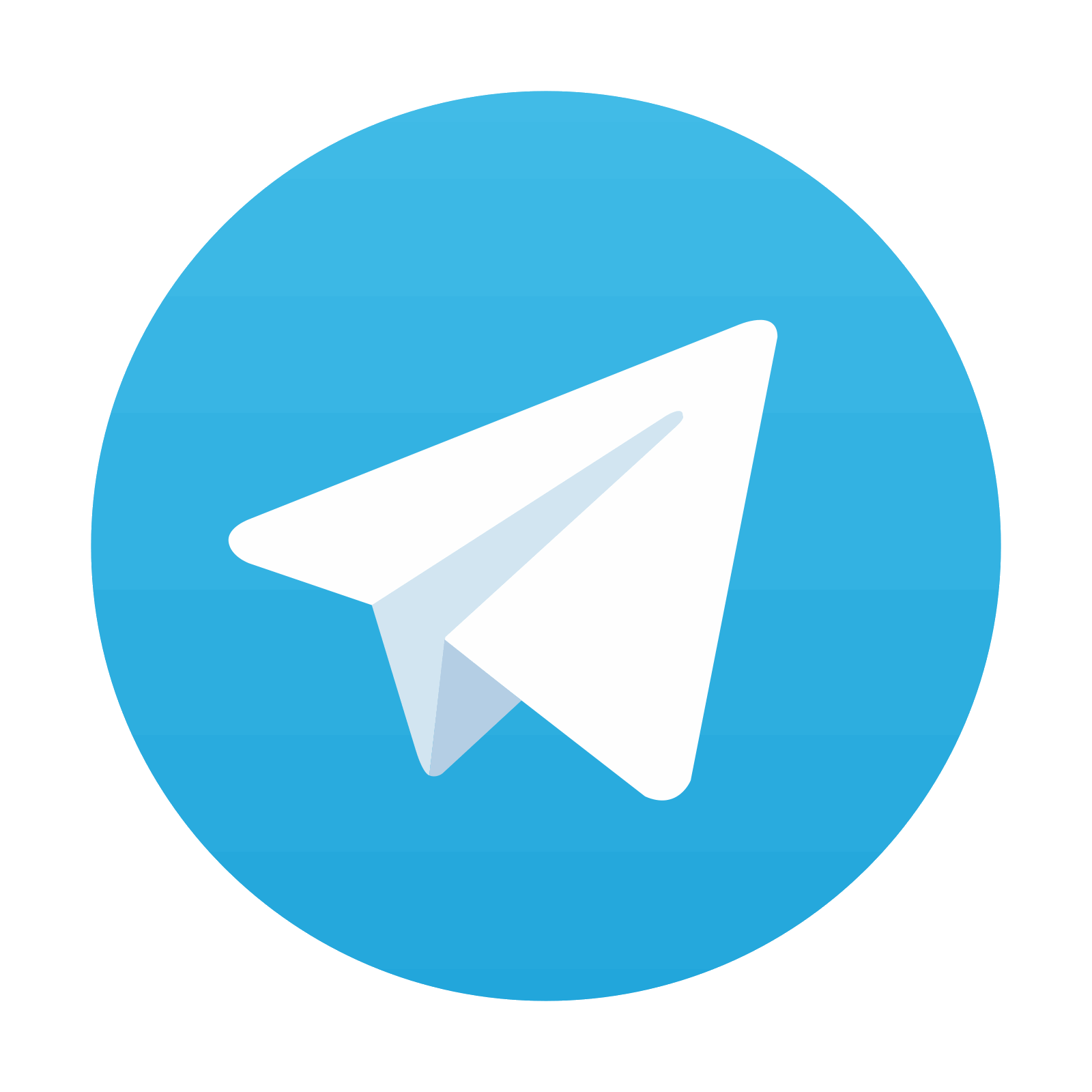
Stay updated, free dental videos. Join our Telegram channel

VIDEdental - Online dental courses
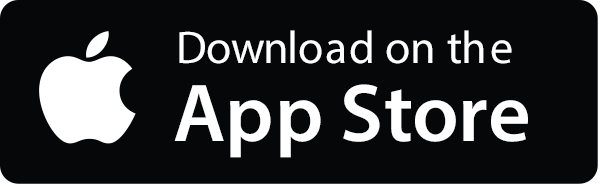
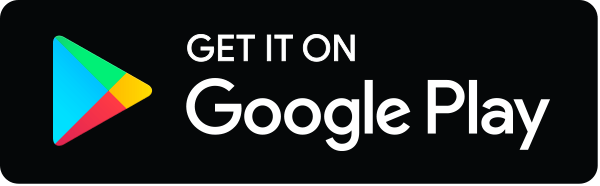