Source
In vitro multipotency
In vivo ectopic tissue formation
DPSCs [7]
Pulp adult teeth
Osteogenic, dentinogenic, adipogenic, chondrogenic, neurogenic, myogenic, cardiomyogenic, hepatocyte-like cell, melanocyte
Dentin-pulp-like complex
Bone-like tissues
Adipose
Muscle
SHEDs [57]
Pulp deciduous teeth
Osteogenic, dentinogenic, adipogenic, chondrogenic, neurogenic, myogenic, bone induction, endothelial cell
Pulp-like tissues
Bone-like tissues
SCAP [67]
apical papilla
Osteogenic, dentinogenic, adipogenic, chondrogenic, neurogenic, hepatocyte-like cell
Dentin-pulp like complex
Bone-like tissues
Dental follicle
Cementogenic, osteogenic, adipogenic, chondrogenic, neurogenic, hepatocyte-like cell
Periodontal tissues (alveolar bone, PDL, cementum)
PDLSCs [84]
Periodontal ligament
Osteogenic, cementogenic, adipogenic, chondrogenic, neurogenic
Periodontal tissues (cementum, PDL)
GMSCs [96]
Gingiva
Adipogenic, chondrogenic, osteogenic, neurogenic, endoderm cell
Cartilage
Bone
Muscle
ABMSCs [99]
Alveolar bone
Adipogenic, chondrogenic, osteogenic
Bone
TPSCs [75]
Dental mesenchyme
Adipogenic, chondrogenic, osteogenic, odontogenic, neurogenic, hepatogenic, endothelial cell
Bone
Dental Stem Cells in Craniofacial Bone Healing
The craniofacial structures constitute the epitome of the vertebrate development as they consist of an incongruous medley of tissues derived from the coordinated integration of each germ layer tissue (i.e., ectoderm, mesoderm, and endoderm) [103]. For example, the anterior section of the calvaria originates from neural crest cells and the parietal bones from mesodermal cells . Specifically, the craniofacial mesenchymal tissues originate from the neural crest, paraxial and lateral mesoderm, with the contribution of the cranial neural crest being the most dominant [104]. Indeed, when the cranial neural crest cells migrate superficially, they demonstrate a stounding multilineage differentiation potential towards cells that generate the majority of the components of the craniofacial complex. Whether the neural crest or mesodermal origin of a bone is related to its function and healing potential remains elusive. However there are convincing insights that the two cell populations exhibit molecular differences that are reflected to their osteogenic potential and regenerative capacity [105, 106]. Further investigation is needed to delineate the possible involvement of these embryological differences to the healing capacity of the discrete craniofacial parts.
Calvarial Bone Defects
Experimental Models of Calvarial Bone Defects
Bone tissue reconstruction in the craniofacial area is typically required in cases of trauma, skeletal diseases, congenital malformations and cancer surgery and affects the life quality of millions of patients worldwide [107]. The ‘gold standard’ bone grafts , despite their associated limitations (autogenous, allogenous, xenogenous and synthetic) [108], constitute the basic therapeutic modalities. The recently introduced treatment alternatives are based on regenerative medicine and bone tissue engineering [109, 110]
Irrespective of the treatment choice, designing appropriate experimental models to evaluate the efficacy and safety of any regenerative procedure stands out as an absolute necessity. In vivo animal models could provide a link between in vitro studies and clinical trials. Although a single ideal model is currently unavailable, there are specific criteria that a model used for bone tissue engineering should meet [111]. The calvarial bone defect in rodents is frequently used as anorthotopic model to evaluate bone regeneration , as it fulfills the basic requirements of an adequate experimental design [112]. Specifically, the rodent calvarial defect model constitutes a uniform, reproducible and standardized defect that allows radiographic and histological analysis; calvaria is an easily accessible site providing sufficient area for surgical handling; the dura mater and the overlying skin provide adequate support for the grafts without the need of any kind of fixation; includes both cortical and cancellous bone; it is inexpensive and widely used, thus allowing for direct comparisons between the different graft materials; it has a fast healing time [113–116].
An important parameter in the design of the calvarial bone defect is its size. The most widely accepted defect size in assessing the osteogenic capacity of different grafts is the critical-size defect (CSD) [117]. CSDs were originally defined as “the smallest size intraosseous wound in a particular bone and species of animal that will not heal spontaneously during the lifetime of the animal” [118] and were later established at the calvaria of rats as the 8 mm [119] or the paired 5 mm cranial defect [120] (see also Fig. 1). Although the value of the CSDs has been keenly criticized [122], it still remains the most encountered osseous defect in the literature and constitutes the ‘gold standard’ experimental model in appraising craniofacial regeneration .
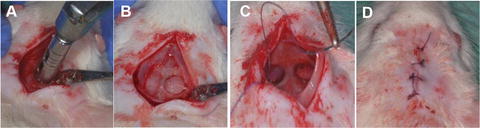
Fig. 1
Surgical procedure in calvaria defects. (a) Preparation of the surgical area. (b) Creation of the 5-mm critical size defect in the rat calvarium. (c) Closure of the periosteum. (d) Closure of the overlaying skin [121]. Reproduced upon permission from Elsevier
Dental Stem Cells and Bone Repair in the Calvarial Experimental Model
The rodent calvarial bone defect model has been employed extensively as an orthotopic experimental design to assess the bone regeneration capacity of the DPSCs in the craniofacial complex. Following the first isolation of MSCs derived from the dental tissues [7], a huge impetus towards the extensive study of their stem cell properties has been noted [69]. Within the context of craniofacial bone engineering, DPSCs constitute a favorable population of adult MSCs for several reasons: they have common developmental origin from the cranial neural crest with the craniofacial bones [123]; they are easily harvested; they exhibit enhanced osteogenic potential in vitro and in vivo compared to other MSC populations [10, 12, 44, 46] and they retain their ‘stemness’ after cryopreservation [54, 56].
As mentioned previously, a series of in vitro studies have clearly demonstrated the capacity of the c-kit+/CD34+/CD45− sub-population from the mixed DPSCs (SBP-DPSCs) to produce two- and three-dimensional structures of mature bone [19, 55, 124]. Further research on the regenerative efficacy of a selected subpopulation of DPSCs (cells positive for c-kit+/CD34+/STRO-1+) seeded on collagen or fibroin scaffolds, has demonstrated exceptional bone healing of critical-size calvaria defects in immunosuppressed rats. The human cells were detected in the areas of newly-formed bone and the enhanced bone healing was attributed also to the increased angiogenic potential of the selected stem cell populations [50, 125, 126]. Similar results were obtained recently with a selected CD271low+/CD90high+ DPSC population. These cells mixed with Matrigel Matrix and implanted into critical-size calvaria defects in immunodeficient mice survived throughout a 4-week period and promoted significantly bone regeneration [47]. These studies have shown that selective DPSC subpopulations, with enriched osteogenic and angiogenic potential, are of utmost importance for an optimal result. The healing properties of the entire population of human dental pulp cells have also been demonstrated in the rat calvaria model [121]. In our study, dental pulp cells isolated from human third molars were transplanted in the 5 mm calvaria defects of immunocompetent rats following a short osteoinduction period in culture. An ECM-mimicking hyaluronic-based scaffold was used as the cell carrier. Enhanced bone regeneration was detected by histomorphometry in the cell-treated defects, compared to the scaffold-treated and untreated ones (Fig. 2). However, in contrast to the complete bone healing observed in the afore-mentioned studies [50, 125, 126], none of the defects exhibited complete bone regeneration in our study. The mechanisms underlying this incomplete bone regeneration may relate to several factors, including the lower osteo/angiogenic potential of the mixed pulp population used and the type and properties of the scaffold employed that was not degraded properly, thus entrapping many of the pulp cells in it. The latter resulted in inflammatory reactions around the non-degraded scaffold islets that contained dead pulp cells (Fig. 2). Though inflammatory signals have a stimulating role in the beginning of the healing process, the host’s response that was detected at a later stage due to pulp cells’ entrapment might have hampered the repair procedure. Despite the incomplete bone restoration, the significantly higher percent of new bone formation in the cell-treated group of this study, compared to the control groups, support the osteogenic efficacy of the entire dental pulp cell population. At the same time, these results underline the need for further improvement of our knowledge on the scaffolds’ in vivo properties and bio-behaviors.
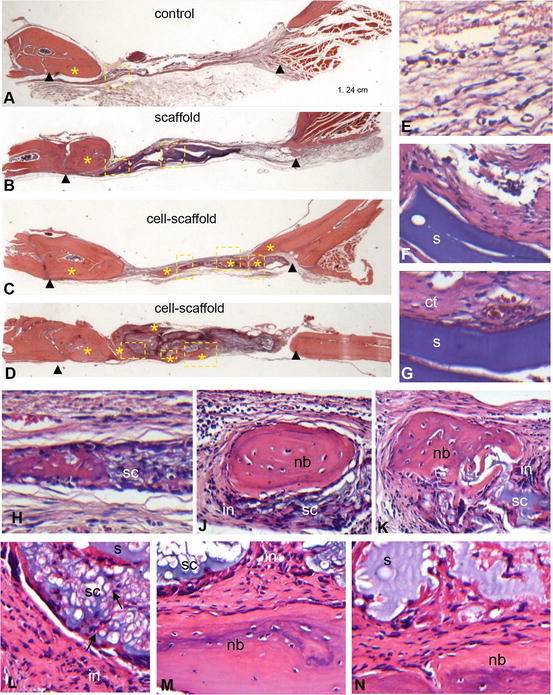
Fig. 2
Histological findings in haematoxylin and eosin stained calvaria sections. Representative low magnification (×1.25) photomicrographs of the defect site in the control (a), scaffold (b) and cell-scaffold (c, d) groups. Arrowheads indicate defect margins. Areas of new bone formation are marked by asterisks. Rectangles indicate sites within defect for which higher magnification (×20) images (e–n) are provided. (e): connective tissue (ct) in the framed area of the control group; (f, g): large areas of scaffold (s) remnants surrounded by dense connective tissue in the framed area of the scaffold group; (h–n): non-degraded scaffold with entrapped cells (sc), and sites of new bone (nb) formation within the defect in the framed areas of the cell-scaffold group in C (h–k) and D (l–n). Note the inflammation (in) around areas of cell-scaffold remnants and dead cells into the scaffold (arrows in L) [121]. Reproduced upon permission from Elsevier
Stem cell-based bone regeneration is critically relayed on the properties of the stem cell populations used. These properties mostly refer to their osteogenic potential. However, additional characteristics may contribute to their efficiency in bone healing, such as their interplay with the host tissue immune response [127] and their paracrine ‘trophic activity’ to adjacent cells [128]. Although the bone regenerative properties of DPSCs in vivo have been shown in the calvarial defects of immunosuppressed rats [50, 125, 126], still little is known about the sequence of events following implantation of the DPSCs. More specifically, studies investigating the biological cues in the defect area after the implantation of the biocomplexes are lacking. The DPSCs-induced immunological response and the paracrine action of the DPSCs warrants for further investigation .
Another important parameter that needs to be taken into consideration in bone regeneration is the type of scaffold employed. These three-dimensional matrices accommodate the dental stem cell populations by forming biocomplexes. The constructs are either cultured in vitro leading to the ex vivo production of bone that is subsequently implanted in the osseous defect or they are implanted directly to the site inducing in vivo bone regeneration [129]. In general, the types of scaffolds used to regenerate calvaria bones can be divided into ceramics and polymers (synthetic or natural) and it is noteworthy that the cell-scaffold interactions as well as the interplay between the bioengineered construct and the host tissue can profoundly affect bone regeneration [130]. In a study assessing calvaria bone regeneration after the transplantation of BMSCs -loaded collagen-glycozaminoglycan constructs in rodents, the initial inflammation induced by the biocomplex led to incomplete bone healing [131, 132]. According to the authors, this host response accelerated the degradation of the scaffold, which in turn impaired the bone matrix deposition [132]. In our study, the utterly different host response to the dental pulp cell-biocomplexes, compared to the inflammatory response towards the cell-free scaffolds, was the major cause of the non-optimal repair of the defects [121].
Besides from DPSCs, stem cells from exfoliated deciduous teeth (SHEDs) have also been successfully used to regenerate critical size bone defects in the calvaria rat model [133–135]. Similarly to the DPSCs, the neural crest origin of the SHEDs and their enhanced stemness make them excellent candidates for craniofacial bone tissue engineering.
Bioengineered constructs comprised of stem cells from the periodontal ligament (PDLCs) have been used to regenerate bone in calvarial defects with very encouraging results, thus rendering this treatment modality a viable option in the field of craniofacial bone regeneration [98, 136, 137].
Finally, stem cells from the dental follicle (DFSCs) have shown enhanced bone formation when transferred as scaffoldless transplants in 8 mm critical-size calvarial defects of immunodeficient rats [138].
Overall, the use of dental stem cells in craniofacial bone engineering seems justified. In particular, the results from the transplantation of DPSCs in the calvarial experimental model render this stem cell-based treatment a quite promising future treatment modality for repairing defects in the craniofacial area. Alternatively, stem cell populations from other dental tissues displaying enhanced osteogenic capacity and sharing common developmental origin with the craniofacial bone structures may also prove beneficial in the cranial bone tissue engineering.
Mandibular and Alveolar Bone Defects
Mandibular bone defects due to traumas, neoplastic tumors or other pathological conditions are challenging problems for oral and maxillofacial surgeons. In most cases, repair of such defects requires bone grafts for restoration of continuity, function and aesthetics. In dentistry, bone grafts are mainly applied in order to repair alveolar bone defects caused by periodontal or peri-implant disease and to preserve or reconstruct the alveolar ridge for future implant placement. Dental stem cells and tissue engineering technology in oral and maxillofacial region stand out as new alternatives for repairing mandibular and alveolar defects.
Experimental Models of Mandibular and Alveolar Bone Defects
The majority of in vivo studies evaluating the application of stem cells of dental origin on mandible defects are performed in experimental animals . Various animal models of mandibular bone defects have been used. Dogs, pigs, rabbits and rats are the most frequently used animals in this field, with dogs and pigs possessing anatomical and physiological features more close to the human ones [139]. The rat model, on the other hand, is dissimilar in terms of size and bone structure compared to humans [36], but is easily handled and cost effective [112].
The type of the mandible defect varies from segmental [140] or cylindrical [141] alveolar defects to orofacial defects in the parasymphyseal region of the mandible [142] or in front of the mandibular corner [143]. All of the above defects are considered critical size defects, in which by definition complete bone healing does not spontaneously occur and therapeutical intervention is needed [118]. The efficacy of dental stem cells on bone regeneration has also been tested in a mandibular distraction osteogenesis model in rabbits [144]. In the clinical setting, alveolar defects of the mandible, produced after extraction of impacted third molars, have been treated with autologous DPSCs seeded onto collagen sponge scaffolds [145].
Alveolar defects of the mandible often linked with periodontal degeneration may result in loss of cementum, periodontal ligament and alveolar bone. Animal models simulating these conditions have been also used for evaluating the regenerative efficacy of dental stem cells. Experimental models of periodontitis are surgically created and can be classified to chronic, acute and acute/chronic ones [146]. Models of chronic defects arise from the reproduction of conditions that induce periodontal tissue loss, such as the placement of orthodontic elastics around the teeth at or slightly apical to the gingival margin [147]. The models of acute defects are created by surgical removal of bone, cementum and periodontal ligament, while the insertion of a foreign body in the surgically created acute defect leads to an acute/chronic defect. The type of acute defect displays heterogeneity; furcation defects [148], intrabony defects in the mesial or distal aspect of the tooth [149] or fenestration defects [150] have been used. Acute defect models have been used for testing bone regenerative properties of dental stem cells, due to their cost-effectiveness and the reduction of experimental time. Regarding the acute/chronic defect, Liu et al. [151] created such defects in the mesial region of mandibular first molars of pigs by surgical removal of bone and subsequent silk ligament suture. Similarly, Park et al. [152] created surgically circumferential defects in the mesial root of mandibular premolars of dogs, which were then filled with a rubber base impression material. The acute/chronic model resembles ‘true’ periodontitis and is the one recommended in periodontal regeneration research. In cases of bone regeneration around dental implants, experimental models in dogs have been used to examine the effect of dental stem cells on peri-implant bone regeneration [153–155].
Unfortunately, there is no ideal experimental model and the selection of appropriate animal and defect model for each clinical application is complicated [146]. The establishment of reliable and validated animal models is necessary for evaluating and comparing the effect of stem cell-based therapies on mandibular bone regeneration .
Dental Stem Cells in the Repair of Mandibular and Alveolar Defects
In addition to the proven in vitro and ectopic in vivo osteogenic properties of DPSCs and SHEDs, these cells exhibit great regenerative capacity when transplanted orthotopically in critical-sized mandibular defects. Dental pulp stem cells from deciduous or permanent teeth, enriched with platelet rich plasma (PRP), enhanced bone formation in cylindrical alveolar defects of the mandible in dogs [140–143]. Importantly, the stem cells of dental origin showed bone regeneration capacity similar to that of BMSCs, which have been considered the gold standard in bone regenerative medicine [141].
Growth factors, scaffolds and stem cells, constitute the three key elements for bone tissue engineering. Growth factors, such as the recombinant human bone morphogenetic protein-2 (rhBMP-2,) promote the osteogenic capacity of the DPSCs. Autologous DPSCs combined with rhBMP-2 induced more new bone formation than even the autologous bone, when engrafted in segmental alveolar defects in rabbits [140]. The osteogenic properties of DPSCs appear to be scaffold-dependent. The resorption rate of the scaffold material is of paramount importance and, thus, mixing DPSCs into biomaterials with suitable resorption rates creates a synergetic effect that enhances bone regeneration [143].
In the only human study so far, autologous DPSCs seeded onto collagen sponge scaffolds successfully repaired an alveolar mandibular defect produced after extraction of an impacted third molar [145]. A three-year follow-up study revealed that the regenerated bone was uniformly vascularized and qualitatively compact, though differed from the physiological cancellous (spongy) bone [45]. The formation of compact bone by the DPSC-grafts is alleged to have positive clinical impact, favoring implant stability and rehabilitation of large bone defects in oral cancer patients [36, 45]. However, this alteration in the regenerated bone type raises some concern on the possible diversion of the transplanted DPSCs’ fate towards undesirable cells and tissues [156]. Further studies are required to assure the absolute control on the behavior of the transplanted multipotent cells in the host environment .
For periodontal tissue regeneration, PDLSCs are considered to be the best dental stem cell type. Several animal studies have evaluated the potential of PDLSCs for regeneration of alveolar periodontal defects and have shown that these cells induce new bone, cementum and periodontal ligament formation in the treated defects [148–152]. In a clinical case series, the application of autologous PDLSCs for the treatment of periodontal defects led to improved periodontal parameters in all cases [157]. DFSCs may be an alternative to PDLCs for periodontal regeneration, although not an equally effective one [152]. Although DPSCs exhibit great bone regeneration capacity, their potential for periodontal regeneration is doubtful due to their limited capacity to form cementum [152, 158]. Instead, SCAP have shown to possess the ability to form PDL-like, cementum-like and bone-like tissues in an in vivo ectopic transplantation rat model [158], but there is lack of evidence regarding their potential to repair periodontal defects. Similarly, there is no sufficient data supporting the use of SHEDs for periodontal regeneration.
As mentioned above, there are few studies evaluating the effect of stem cells of dental origin on peri-implant bone regeneration. PDLSCs have been shown to enhance alveolar bone regeneration in surgically created peri-implant saddle-like defects, although to a lesser extent than BMSCs [154]. Notably, the osseointegration of dental implants, assessed by measuring bone/implant contact, was higher in DPSCs/PRP-treated alveolar defects, compared to analogous BMSCs/PRP-, periosteal cells/PRP-treated and untreated defects [153]. Furthermore, Omori et al. [155] suggested that treatment of Ti implants with SHED -conditioned medium promotes bone morphogenesis not only around the implant interface, but also at distant locations from the implant surface, thus improving early osseointegration.
Dental Stem Cells in Long Bone Healing
During osteogenesis, the long bones of the body are formed by endochondral ossification through distinct steps that include: differentiation of mesenchymal stem cells into chondrocytes; proliferation, hypertrophy and cartilage production by the chondrocytes; differentiation of osteoblasts and bone matrix deposition. In adult bone repair, both endochondral and intramembranous ossification (i.e. without prior cartilage formation) participate in the healing process depending on the degree of fracture stabilization.
The need to enhance the healing of long bone fractures is well appreciated, especially in cases of unsuccessful unions or non-unions. The use of autologous or allogenic bone grafts is the current gold standard. However, this application is accompanied with severe drawbacks including invasiveness, limited graft volume and bone loss at the donor site. Bone tissue engineering , by using isolated bone marrow stem cells in combination with osteoinductive or osteoconductive scaffolds, emerges as a promising alternative to bone transplants. Animal studies in rodents, rabbits, or bigger mammals have used BMSCs to successfully treat long bone fractures [159]. In these models of bone regeneration, BMSCs have been mostly isolated from same-species donors and the stem cells have been used in combination with scaffolds of various types, including calcifying agents, synthetic polymers and ECM-mimicking biomaterials. In some occasions, scaffold-free approaches have also been used, where the cells are applied as multilayered cell sheets produced in culture prior to transplantation [160, 161]. In the majority of the published animal data, the mesenchymal stem cells enhanced the healing process [6]. The ongoing clinical trials in the field, using therapeutic approaches based on the use of bone marrow stem cells/osteoinductive scaffolds have not yet provided published results [162].
The osteogenic properties of DPSCs are quite comparable with those of stromal BMSCs, both in vitro and upon ectopic transplantation [7, 10]. During the last decade DPSCs have been used in animal models of craniofacial bone healing with promising results. However, neither DPSCs nor other stem cells of dental origin have been tested in long bone repair. Given the mesenchymal origin and properties of DPSCs, we were interested to examine the efficacy of dental pulp cells, isolated from human impacted third molars, in the restoration of osteotomized rat tibia. In humans, the dental pulp from impacted immature third molars that are extracted from healthy young subjects is the preferred source of DPSCs. Additionally, dental pulp contains a substantial population of progenitor cells conferring a niche for the ‘true’ stem cells [163]. We therefore used the entire population of dental pulp cells instead of isolating the DPSCs. The presence of typical mesenchymal stem cell markers and the osteo-differentiation potential of the entire dental pulp population were verified in vitro by flow cytometry. To avoid reduction of the stemness of the pulp cell population following osteo-differentiation and/or repeated subcultures [164, 165], early passage, undifferentiated, pulp cells were transferred into the fracture site. It was also decided to follow a scaffold-less transfer of the cells in order to clearly detect any stem cell-induced effect. Osteotomies were performed in adult male rats and stabilized with intramedullary nails (Fig. 3). The healing process was monitored radiologically for 8 weeks. Quantification of the X-ray films showed a significantly smaller and more homogenous callus in the cell-treated rats compared to the controls, denoting advanced bone healing in the former group. The radiological results were further supported by the histological observations at 8 weeks post surgery [166]. Cell-treated animals exhibited a remodeled bone marrow cavity and higher percentage of lamellar new bone, compared to the vehicle-treated ones that had a higher score of non-bridging and scar tissue (Fig. 4).
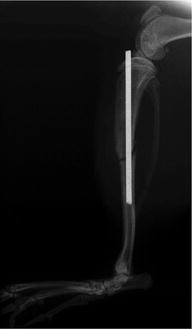
Fig. 3
Representative X-ray of the rat tibia fracture model used in the study of [166]
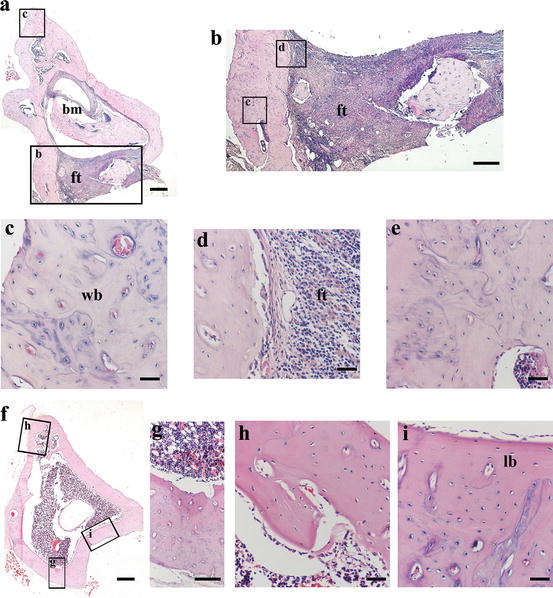
Fig. 4
Representative histological sections stained with haematoxylin and eosin (H&E) from rat tibias at 8 weeks post-fracture of control (a–e) and dental pulp cell-treated (f–i) animals [166]. H&E stained sections show smaller bone marrow cavity (a), non-bridging (a, b), extended fibrous tissue (a, b, d) and mostly woven bone (c, e) in the controls, compared to cell-treated (f–i). Framed areas in a, b and f refer to higher magnification images, according to the letter inside the upper left side of the frame. bm bone marrow, ft fibrous tissue, lb lamellar bone, wb woven bone. Reproduced upon permission from J Sci Med Central
To our knowledge, this is a first approach to examine in vivo the effectiveness of dental pulp cells to heal long bone fractures. The results provide evidence that the whole population of dental pulp cells from adult human dentition can promote bone healing in a rat osteotomy model. The developed model was purposely kept simple (i.e. scaffold-less, without specific cell sorting, without immunosuppression of the host that could potentially hinder healing initiation; [2]), in order to investigate a potential cellular effect. Further refinement of the protocol is necessary to delineate the optimal conditions for the best biological outcome. Additionally, there is need for detailed characterization of the cellular interactions (including dental pulp cells’ fate in the host tissue) and the molecular responses during the process of bore repair .
Conclusion and Future Perspectives
For successful cell-based bone repair, the candidate stem/progenitor cell population must fulfill the following key criteria: (a) to differentiate into bone forming cells or to stimulate endogenous tissue repair, (b) to impede uncontrolled growth and differentiation, (c) to be tolerated by the host immune system, (d) to support neoangiogenesis and blood supply, (e) to form biocomplexes with scaffolding materials, (f) to be easily harvested in a harmless manner, (g) to retain stemness upon cryopreservation and (h) to be cost effective.
Experimental evidence from mesenchymal stem cells of dental origin (DSCs) suggests that several types of these stem cells can satisfy the above criteria. Indeed, they are responsive to osteogenic signals and exhibit a bone forming potential comparable to that of BMSCs. Their origin (adult mesenchymal stem cells) assures a minimal oncogenic potential compared to pluripotent cells. DSCs are well tolerated in the fracture site and some categories have immunosuppressive properties. Discrete subpopulations of DSCs have been shown to promote new vessel formation at the fracture site. Most types of DSCs can form composite grafts with several types of scaffold materials. Importantly, they can be obtained in a non-invasive and low cost procedure and retain their osteogenic properties upon storage.
The results from the use of DSCs in animal models and the one human study of bone repair are encouraging, especially for the restoration of craniofacial bone defects. However, the existence of different models of bone repair in combination with the different subpopulations of DSCs and scaffolds used, has not allowed all proper comparisons. More studies are thus required to define the most valuable healing approach in terms of the bone defect, and the cell population/scaffold used. Furthermore, there is need to expand our knowledge on the biobehavior of the transplanted cells in the in vivo situation, regarding their interaction with the carrier material, their cell fate and possible induction properties.
References
1.
Deschaseaux F, Sensébé L, Heymann D (2009) Mechanisms of bone repair and regeneration. Trends Mol Med 15(9):417–429PubMed
2.
Schmidt-Bleek K, Schell H, Schulz N, Hoff P, Perka C, Buttgereit F, Volk HD, Lienau J, Duda GN (2012) Inflammatory phase of bone healing initiates the regenerative healing cascade. Cell Tissue Res 347:567–573PubMed
3.
Friedenstein AJ, Chailakhjan RK, Lalykina KS (1970) The development of fibroblast colonies in monolayer cultures of guinea-pig bone marrow and spleen cells. Cell Tissue Kinet 3(4):393–403PubMed
4.
Friedenstein AJ, Piatetzky S II, Petrokova KV (1966) Osteogenesis in transplants of bone marrow cells. J Embryol Exp Morphol 16(3):381–390PubMed
5.
Rosset P, Deschaseaux F, Layrolle P (2014) Cell therapy for bone repair. Orthop Traumatol Surg Res 100:S107–S112PubMed
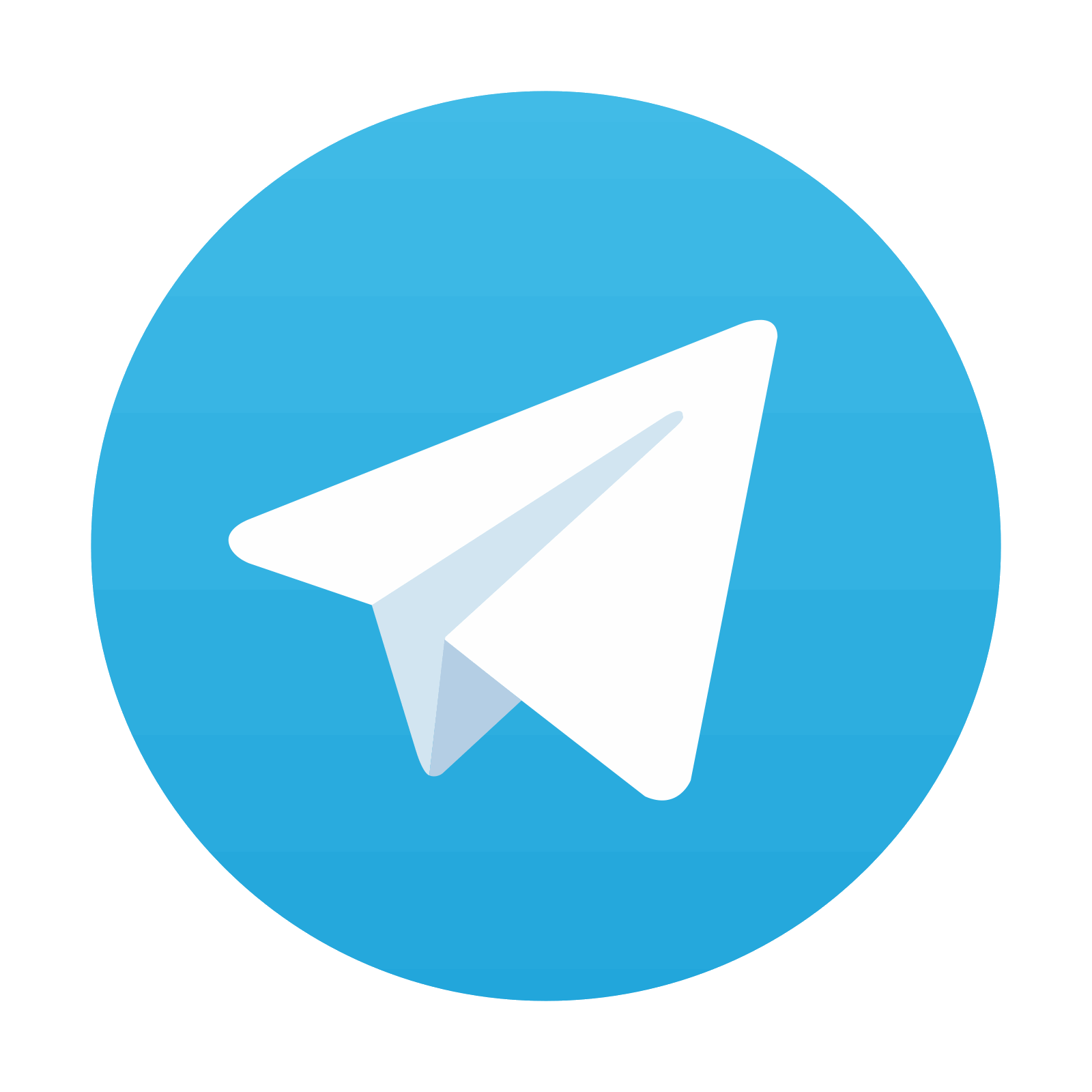
Stay updated, free dental videos. Join our Telegram channel

VIDEdental - Online dental courses
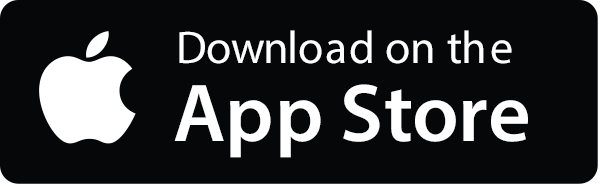
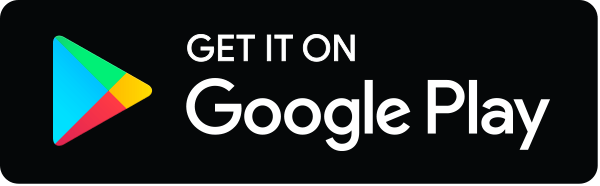