Fig. 1
Developmental potential of DPSC transplanted with HA/TCP carrier in vivo . (a) After 6 weeks, DPSC generated a dental pulp-like tissue within the HA/TCP carrier (c) with the presence of dentin-like matrix (d), odontoblast-like cells (od) and blood vessels (bv). (b) A magnified view of the dentin matrix (d) highlights the odontoblast-like layer (od) and odontoblast processes (arrow) (c). Polarized light demonstrates perpendicular alignment (dashed lines) of the collagen fibers to the forming surface. Adapted from Gronthos et al. [31] (Copyright (2000) National Academy of Sciences, U.S.A.)
The residual pulp of exfoliated deciduous teeth contains stem cells (SHED) that can regenerate a dental pulp-like tissue that can deposit mineralized tissue [6, 22, 27, 45]. These cells showed their potential to be used for dental pulp tissue regeneration in 2003 in a seminal paper published by Miura et al. There, SHED mixed with HA/TCP were capable to differentiate into odontoblasts after 8 weeks from implantation in immunocompromised mice. Nonetheless, the cells failed to regenerate a complete dentin–pulp-like complex in vivo as previously observed for DPSCs (Fig. 2) [22, 31]. Nonetheless, this ability was reported later [6, 27, 59].

Fig. 2
(a) After 8 weeks of transplantation, SHED were able to differentiate into odontoblasts (open arrows) that were responsible for the dentin-like structure (D) formation on the surfaces of the HA/TCP scaffold (HA). (b) In situ hybridization showed that the tissue formed had human origin (open arrows). (c) The black dashed line represents interface between newly formed dentin. (d) bone (B) generated by host cells in the same SHED transplant shows no reactivity to the DSPP antibody. Adapted from Miura et al. [22] (Copyright (2003) National Academy of Sciences, U.S.A.)
Although SCAP is one of the candidates to be used in dental pulp tissue engineering, the majority of the research for such purpose focus on the use of stem cells obtained from the dental pulp itself (DPSC and SHED). Although SCAP are capable of forming odontoblast-like cells to produce dentin in vivo, they are likely to be the source of primary odontoblasts for the root dentin formation. This limits their isolation to the period when the root is not yet fully formed [24]. Thus, third molars are the most attractive source of SCAP as their root completion is only achieved by ages from 18 to 25. DPSC can be obtained from the dental pulp of permanent tooth. Here, pre-molars that are extracted due to orthodontic reasons are also an interesting source of DPSC. SHED can be retrieved from the only naturally disposable post-natal human tissue. This creates a window of approximately 11 years to obtain these stem cells mainly from deciduous central incisors and canines.
The success of cell-based regenerative endodontic strategies depends on techniques that will allow clinicians to create a functional pulp tissue within cleaned and shaped root canal systems [5, 17, 20, 52]. One of the first signs that this could be achieved was reported in 1996. The team led by David Mooney engineered pulp-like tissues in vitro utilizing fibroblast obtained from human adult dental pulp seeded in a synthetic extracellular matrix made of polyglycolic acid fiber-based meshes. Histological analysis performed after 60 days from implantation showed that the cells were capable to proliferate and form a new tissue with general appearance and cell density similar to native dental pulp [60]. In 2004, autogenous transplantation of pulp cells stimulated with bone morphogenetic protein 2 (BMP-2) into amputated pulp of dogs induced the formation of reparative dentin formation [61]. These and other studies shed a light that cell-based regenerative endodontics could be on the horizon of clinicians in the future. Since then, a series of proof-of principles research have been published showing the feasibility of regenerating functional human dental pulp using tissue engineering principles and dental stem cells.
To prove the potential of dental stem cells to induce pulp regeneration in vivo, several reports use the Tooth Slice/Scaffold of Dental Pulp Tissue Engineering model . This strategy allows for the generation of a dental-pulp-like tissue via the transplantation of human dental pulp stem cells seeded in a biodegradable scaffold cast within the pulp chamber of human tooth slices [62]. This model was used to show the ability of SHED to generate dental pulp-like tissue in vivo by the Nör’s lab in 1996. There, SHED were seeded into poly-l-lactic acid (PLLA) scaffolds cast in 1 mm thick tooth slice and transplanted into the subcutaneous space of immunocompromised mice. After 4 weeks, a well-vascularized pulp-like tissue was formed in the pulp chamber space. Notably, there was a layer of odontoblast-like cells expressing dentin sialoprotein (DSP) lining the dentin surface. Although these cells were morphologically similar to odontoblasts, including the eccentric polarized position of the nucleus at the basal part of the cell body, no new dentin deposition was observed [46].
The capability of SHED to differentiate into fully functional odontoblasts capable of generating tubular dentin in vivo was observed later (Fig. 3). For this, SHED were seeded a poly-l-lactic acid (PLLA) scaffold cast in a tooth slice and implanted subcutaneously into the dorsum of mice, which received one injection of tetracycline hydrochloride every 5 days. After 32 days from implantation, the dentin disks presented well-defined fluorescent lines originated from the chelation of calcium ions in the newly deposited dentin. The dental pulp generated displayed positive protein expression for dentin sialophosphoprotein (DSPP) and dentin matrix protein 1 (DMP-1) . Interestingly, the rate of dentin formation by SHED was far superior (14.1 μm/day) as compared to the one observed for the human dental pulp used as control (3.3 μm/day) [27]. Growth rates of 4–15 μm/day have been reported for primary dentin depending on the stage of development and age of the tooth [63].
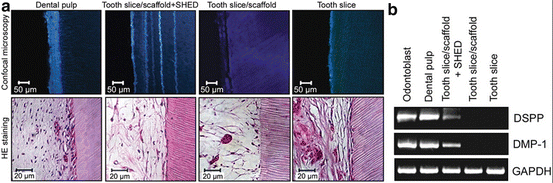
Fig. 3
SHED differentiate into functional odontoblasts that generate new tubular dentin in vivo. (a) The pictures show samples retrieved from mice after 32 days after implantation. (b) RT-PCR analysis showed the high expression of DSPP and DMP-1 on tissues engineered on tooth slice/scaffolds seeded with SHED. Reprinted from Journal of Dental Research, 89/8, VT Sakai, Z Zhang, Z Dong, KG Neiva, MA Machado, S Shi, CF Santos and JE Nör, SHED differentiate into functional odontoblasts and endothelium, 791–796, Copyright (2010), with permission from SAGE Publications
DPSC were also used to regenerate pulp-like tissue using a similar approach. By seeding DPSC in a collagen-based scaffold along with a growth-factor (DMP-1) it was possible to engineer pulp-like tissue after 6 weeks from subcutaneous transplantation in mice. However, in this study, no odontoblast-like cells were found on the surface of pre-existing dentin surface and the quality of the pulp-like tissue obtained appeared to be less promising as the ones obtained using PLLA scaffold [64]. These drawbacks can be related to the contraction that collagen scaffold experiences leading to a size reduction proportional to the density of cells seeded in it [65].
Although these studies provide an exciting panorama for regenerative endodontics, the use of tooth substrates with few millimetres in thickness does not fully represent the challenges of regenerating a pulp tissue with in complete roots [6]. The larger dimension of the tissue that has to be engineered in addition to the fact that vascularization can be available only through the apex, pose important obstacles to be overcome. Hence, some studies aimed to prove that pulp regeneration can be achieved in three-dimensional environments.
An important step towards stem cells-based regenerative endodontics was obtained by a team of researchers who have seeded swine DPSC into the root segments with 5–6 mm in length and implanted into the jawbone of the adult minipigs. After 6 weeks from implantation, the proliferating cells were filling the space once left by the scaffolds made of collagen or poly(lactic-co-glycolic) acid (PLGA) undergoing degradation. At that time, new extracellular matrix had been occasionally deposited in a polar predentin-like pattern on the canal dentinal walls. After 10 weeks, a continuous layer of cells with columnar or spindle-shaped morphology along with the presence of newly formed organic matrix was observed [66]. An interesting fact about this study was that the swine DPSC used were cryopreserved for 1 year prior to implantation showing that the DPSC do not lose their potential to regenerate dentin/dental pulp-like tissue even after being cryopreserved.
Dental pulp tissue formation using DPSC was also achieved within tooth slices as long as 7 mm in length. Cells mixed with poly-d, l-lactide and glycolide (PLG) scaffolds were inserted into the canal with internal space ranging from 1 to 2.5 mm and one end sealed with MTA. Four months after the implantation into the subcutaneous of mice, the emptied canal was filled with regenerated pulp-like tissue. The entire pulp-like tissue was vascularized with a uniform cell density resembling the natural pulp. Though the odontoblast-like cells were not well organized and did not present characteristics of natural odontoblasts, a layer of mineralized tissue was deposited onto the dentinal walls and the MTA cement used to seal the canal [67]. However, as MTA is known to release calcium ions that induce the formation of calcified barriers [68] it was not possible to determine if DPSC alone could induce such extent of calcified tissue deposition. Nonetheless, that paper offered great perspectives on the capability of using dental stem cells to induce de novo synthesis of vascularized human pulp-like tissue capable to deposit dentin-like tissue onto the surface of root canals [67].
SHED are also capable to regenerate dental pulp within full-length root canals in vivo (Fig. 4). Cells were injected in empty roots using either a self-assembling hydrogel or recombinant human collagen type I. The roots were implanted in the subcutaneous of mice and after 28 days a human pulp-like tissue was observed throughout the extent of the root canals. More excitingly, the engineered pulp was capable of generating new dentin at a rate of approximately 10 μm/day. The high activity of the functional odontoblasts was supported by a high concentration of blood vessels close to the dentinal walls (Fig. 4b) [6]. One fact that deserves to be highlighted in this study is that cells were delivered inside the root canals using injectable scaffolds . As injection is one the most practiced procedures by dentists, the learning curve can be shortened for clinicians to apply this technology in patients.
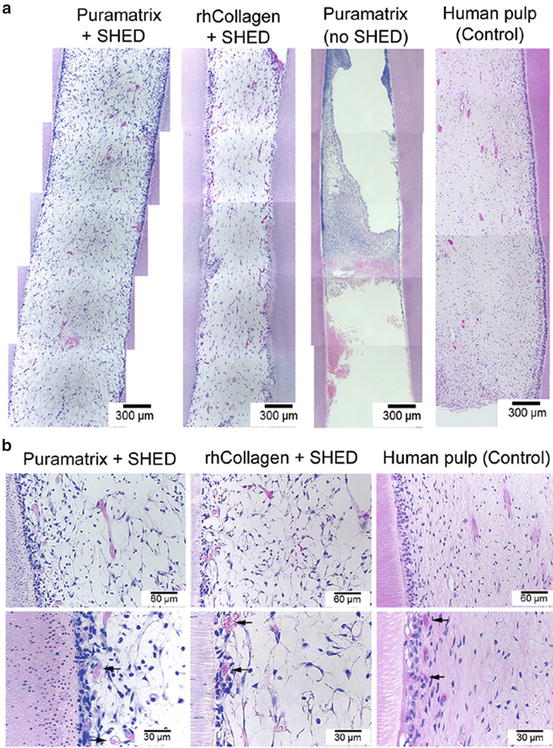
Fig. 4
Dental pulp tissue engineering with SHED injected into human root canals and transplanted into immunodeficient mice. (a) Low-magnification and (b) high-magnification images of tissues formed when SHED mixed with scaffolds (Puramatrix™, rhCollagen type I groups) were injected into full-length root canals of human premolars. A vascularized connective tissue occupied the full extension of the root canal. Cell densification and many blood vessels were observed along dentin walls. Scaffolds (Puramatrix™) injected into the root canals without cells were used as controls for SHED. Freshly extracted human premolars were used as tissue controls. Black arrows point to blood vessels close to the odontoblastic layer. Reprinted from Journal of Dental Research, 92/11, V Rosa, Z Zhang, RHM Grande and JE Nör, Dental Pulp Tissue Engineering in Full-length Human Root Canals, 970–975, Copyright (2013), with permission from SAGE Publications
Future Challenges
Stem cells-based therapies offer new perspectives targeting dental pulp regeneration and further development of root structure [17]. Besides that, the two branches of the tissue engineering triad (the development of suitable scaffolds to deliver cells and fine tuning of signalling pathways) still offer several challanges to be solved in order to improve the outcomes.
Scaffolds are frameworks that provide the support for cells to proliferate, differentiate and generate the desired tissue [5, 6]. Ideally, a scaffold must allow cell attachment and migration, permit the localized and sustained delivery of growth factors and enable the influx of oxygen to maintain the high metabolic demands of cells and tissues in formation [5].
The scaffolds physical and mechanical characteristics must be compatible with the surrounding tissues to support specific demands in vivo [69–71]. It is widely known that stiffness of the substrate play an important role in differentiation of stem cells thus. In fact, the physical and mechanical properties of the scaffolds can have direct impact in cell differentiation through mechano transduction [5, 69, 72]. Interestingly, the increase of scaffold’ stiffness favours the differentiation of MSCs into neurons, myoblasts, and osteoblasts in that order [72]. DPSC seeded on soft matrices present upregulation of collagen I but downregulation of markers such as DSPP, DMP-1 [73]. In addition, physical features of the scaffolds (e.g. quantity and extension of pores) change the specific surface modifying its permeability and mechanical properties, influencing cell differentiation and tissue formation [5, 25, 74]. Noticeably, higher number and extension of pores can contribute significantly for cellularity but compromise scaffold strength [74, 75]. Pore interconnectivity also plays a crucial role to sustain tissue growth [75, 76]. Also, the rate of scaffold degradation is important to achieve success in tissue engineering therapies. The scaffold should ideally reabsorb once it has served its purpose of providing a template for tissue regeneration. The degradation ought happen at a rate compatible with the new tissue formation [5, 27, 71, 73, 76]. The by-products generated cannot be toxic and must be easily cleared or resorbed to minimize the risk of inflammatory response [77].
The third pillar to be tuned for successful tissue engineering is cell signalling [5, 52]. This is a complex system of communication that governs cell activities individually and it can be dramatically changed upon cellular interactions or external stimulation. This could be observed when proteins present in dentin disks [78], dentin extract in EDTA [79] or a tooth-germ conditioned extract [80] were found to supplement the scaffolds as a mechanism of cellular induction. For example, there is evidence that the TGF-1 is released from the dentin after injuries [81] and dentin itself can induce the odontogenic differentiation by releasing embedded growth factors like transforming growth factor-β1 (TGF-β1) [82]. Moreover, BMP’s are involved in the odontoblastic differentiation processes [59, 83] and both BMP-2 and 7 present inductive effects in reparative dentinogenesis [84, 85]. Nonetheless, SHED undergoing odontoblastic differentiation responds strongly to BMP-2 as compared to BMP-7 [59]. This complex system where molecules can trigger different responses creates a very challenging environment for researchers developing cell-based therapies.
Other challenge that needs to be addressed is the cost of therapies when available at large. Comparatively to routine root canal treatments, regenerative endodontics requires additional procedures and qualified manpower in order to isolate, expand, preserve and prepare cells for use. Undoubtedly, these add extra cost to the process that eventually will be directed to patients preventing popularization of the treatments. There are lots of research efforts to optimize process and minimize the number of procedures impacting the economic burden. History has shown that most of the revolutionary technologies became vertiginously popular as they also became more affordable. Hopefully, this also can be true for tissue engineering in endodontics (Fig. 5).
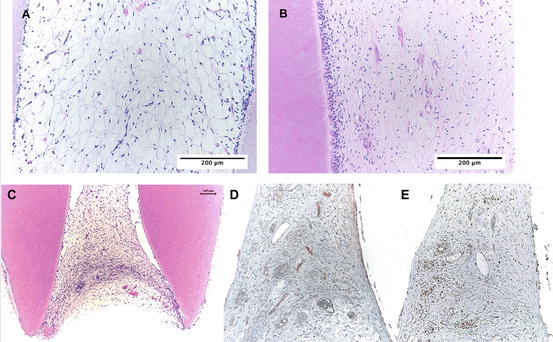
Fig. 5
Dental pulp tissue engineered inside root canal using SHED cells (a) and natural dental pulp from a young premolar (b). The engineered tissue occupies the apical portion (c). Immunohistochemistry with PCNA (proliferating cell nuclear activity) and Factor VIII show a proliferative tissue with established blood network (d, e). Reprinted from Dental Materials, 28/04, B Cavalcanti, A Della Bona and JE Nör, Tissue engineering: From research to dental clinics, 341–348, Copyright (2012), with permission from Elsevier
References
1.
Nanci A (2007) Ten cate’s oral histology-pageburst on vitalsource: development, structure, and function. Elsevier Health Sciences, St. Louis
2.
Huang GTJ (2011) Dental pulp and dentin tissue engineering and regeneration: advancement and challenge. Frontiers in Bioscience (Elite Ed) 3:788–800CrossRef
3.
Zhang W, Yelick PC (2010) Vital pulp therapy—current progress of dental pulp regeneration and revascularization. Int J Dent 2010, 856087CrossRefPubMedPubMedCentral
4.
Ingle JI (2008) Ingle’s endodontics 6. PMPH-USA, Shelton, CT
5.
Rosa V, Della Bona A, Cavalcanti BN, Nor JE (2012) Tissue engineering: from research to dental clinics. Dent Mater 28(4):341–348CrossRefPubMedPubMedCentral
6.
Rosa V, Zhang Z, Grande RH, Nor JE (2013) Dental pulp tissue engineering in full-length human root canals. J Dent Res 92(11):970–975CrossRefPubMedPubMedCentral
7.
Kojima K, Inamoto K, Nagamatsu K, Hara A, Nakata K, Morita I et al (2004) Success rate of endodontic treatment of teeth with vital and nonvital pulps. A meta-analysis. Oral Surg Oral Med Oral Pathol Oral Radiol Endod 97(1):95–99CrossRefPubMed
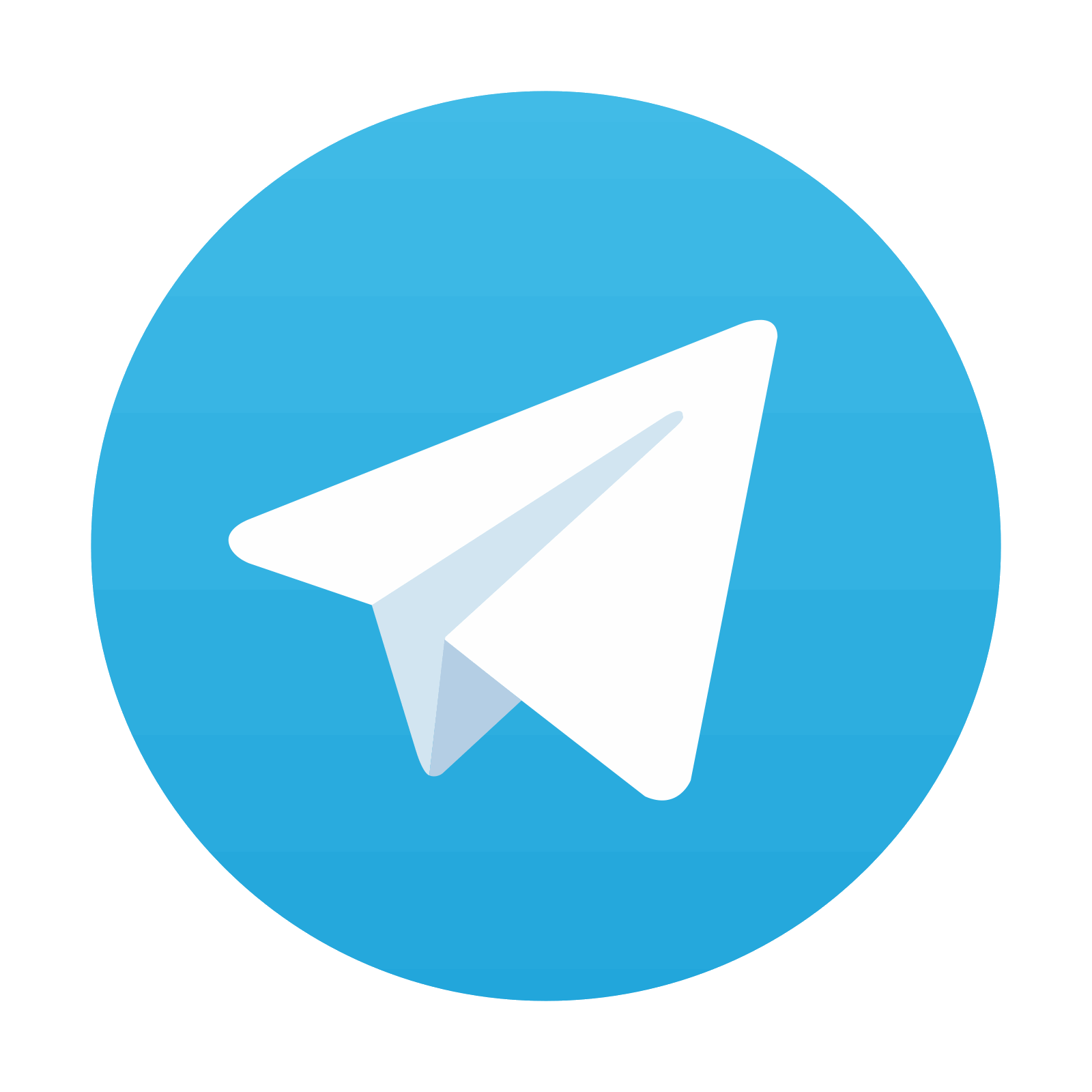
Stay updated, free dental videos. Join our Telegram channel

VIDEdental - Online dental courses
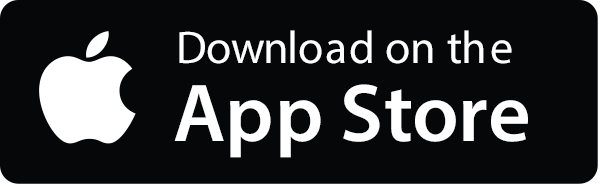
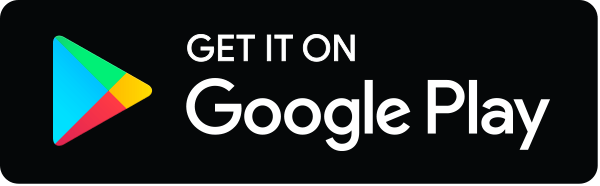