© Springer International Publishing Switzerland 2016
Barbara Zavan and Eriberto Bressan (eds.)Dental Stem Cells: Regenerative PotentialStem Cell Biology and Regenerative Medicine10.1007/978-3-319-33299-4_12
Neuronal Properties of Dental Stem Cells
(1)
Department of Biomedical Sciences, University of Padova, via Ugo Bassi 58/B, 35131 Padova, Italy
(2)
Department of Neurosciences, University of Padova, via Giustiniani 5, 35128 Padova, Italy
Keywords
Neurodegenerative disordersAlzheimer’s diseaseParkinson’s diseaseHuntington’s diseaseMultiple sclerosis
The neurodegenerative disorders are characterized by loss of nerve structure or function. The prevalent disorders are Alzheimer’s disease , frontotemporal dementia , amyotrophic lateral sclerosis, Parkinson’s disease , Huntington’s disease , and multiple sclerosis . These neurodegenerative diseases are increasing worldwide, not only due to increased incidences of direct or indirect injury to the central nervous system (CNS) but also due to the increase in the percentage of the aging population [1]. Despite great progress in understanding the etiology of these disorders, the underlying mechanisms are still indistinct. Furthermore, no means of treating the underlying cause have been devised. For the past decade, researchers have been interested in stem cells and the prospect of using them for understanding the pathogenesis of disease and for facilitating the development of novel therapeutics [2]. In model organisms, both endogenous and exogenous neural stem cells (NSCs) have been investigated for their capacity to regenerate a damaged nervous system [3]. Due to the low incidence of human adult NSCs and problems with accessibility, the use of exogenous sources of stem cells with neural potential has been suggested as a plausible approach to stem cell therapy. Although embryonic stem cells (ESCs) and bone marrow stem cells (BMSCs) have been assessed as potential candidates for neuronal therapy, adult stem cell populations derived from cranial neural crest cells may possess a greater propensity for neuronal differentiation and repair [4]. Interestingly, the dental pulp tissue, termed “ectomesenchyme”, derives from ectodermal cells growing on the periphery of the neural tube during embryonic development, migrating into the oral region, and transdifferentiating into a mesenchymal phenotype [5]. Consequently, Dental Stem Cells (DSCs) have been proposed as a promising source of stem cells to treat nerve regeneration due to their close embryonic origin and easy accessibility. Indeed, DSCs can be obtained from extracted teeth and their surrounding tissue that are usually discarded during dental procedures without invasive methods and additional injury.
Preliminary investigations into the neural potential of human adult DSCs have shown that, under non-neuronal inductive conditions, these cells expressed the neural progenitor marker nestin, and the glial marker glial fibrillary acidic protein (GFAP) , at both the gene and protein levels. The DSCs retain their neural crest properties following ex vivo expansion, and express the postmitotic neuron-specific marker neuronal nuclei (NeuN) , when cultured under neural inductive conditions [4, 6–8]. Therefore, neural crest-derived adult DSCs exposed to the appropriate environmental cues could differentiate into functionally active neurons and could provide an alternative stem cell source for the treatment of neuronal disorders and injury.
The neural differentiation potential of human adult dental pulp stem cells (DPSCs) was demonstrated by Arthur and colleagues [4]. DPSCs were cultured in neuronal inductive conditions with mitogen factors Epidermal Growth Factor (EGF) and basic Fibroblast Growth Factor (FGF) for 3 weeks. Following 3 weeks of induction, DPSCs acquired a bipolar and stellate morphology consistent with that of sensory and motor neurons, respectively. Assessment of the cell proliferation status of DPSCs over the same time period showed that there was a significant decrease in the proliferation rate of DPSCs cultured in neuronal inductive media compared with non-neuronal inductive condition. Immunocytochemical analysis showed that ex vivo expanded DPSCs constitutively express the proteins nestin and GFAP . While nestin protein expression continued to be detected by the majority of DPSCs following neuronal induction, nestin gene transcription was found to be downregulated at 3 weeks after neuronal induction, correlating to neuronal maturation. Also the expression of βIII-tubulin, a neuronal specific marker expressed during early brain development and downregulated in adult brain [9], was evaluated. Whereas βIII-tubulin protein increased over the 3 week induction period, the mRNA level was found to be downregulated, which also correlated to the observed reduction in nestin gene expression. Moreover, the number of DPSCs expressing either neurofilament (NF) medium (NFM) or NF heavy (NFH) gene or protein were found to be significantly higher following neuronal induction compared with non-neuronal inductive condition [4].
Also human exfoliated deciduous teeth (SHED) showed neural differentiation potential. SHED differ from DPSCs with respect to their higher proliferation rate, increased cell-population doublings, sphere-like cell-cluster formation, osteoinductive capacity in vivo, and failure to reconstitute a dentin-pulp-like complex [8]. Therefore, SHED apparently represent a population of multipotent stem cells that are perhaps more immature than previously examined postnatal stromal stem-cell populations. SHED under non-neuronal inductive conditions express a variety of neural cell markers including nestin, βIII-tubulin, glutamic acid decarboxylase (GAD), NeuN, GFAP, NFM, and 2′,3′-cyclic nucleotide-3′-phosphodiesterase (CNPase). After 4 weeks of neural inductive culture, SHED lost their fibroblastic morphology developing multicytoplasmic processes and increased the expression levels of neuronal markers including βIII-tubulin, GAD, and NeuN, whereas the levels of nestin, GFAP, NFM, and CNPase remained unchanged [8]. These findings were confirmed by Govindasamy and co-workers that compared the neural differentiation potential of SHED and DPSCs [10]. They observed that, under non-neuronal inductive conditions, the proliferation rate of SHED was higher than that of DPSCs. The fold expression of several pluripotent markers such as OCT4, SOX2, NANOG, and REX1 were higher in SHED as compared with DPSCs. Conversely, DPSCs showed higher expression of neuro-ectodermal markers PAX6, GBX2, and nestin. These data supported the notion that SHED are more primitive or pluripotent cells than DPSCs. Indeed, the overexpression of the transcription factors OCT4, SOX2, and NANOG are responsible for the maintenance of pluripotency in early embryos and ESCs [11]. However, when SHED and DPSCs were cultured in non-coated dishes containing the neuronal induction factors FGF and EGF for 15 days, both cells were capable of forming distinct neurospheres, in which growing cells aggregate in floating spheres. Once attached in coated dishes, the neurospheres derived from SHED and DPSCs spontaneously showed outgrowth and dendrite-like structure and expressed neuronal markers, such as βIII-tubulin, NF and GFAP. Despite SHED and DPSCs came from the same origin, higher neurosphere formation and neuronal marker expression were found in the differentiated DPSCs into neuron-like cells as compared with SHED. Since nestin is essential for the induction of neurospheres [12], the high level of nestin expressed by undifferentiated DPSCs could potentially enable them to differentiate more efficiently than SHED into neuronal cells. Nevertheless, SHED could also be forced to form neuronal cells under the influence of appropriate microenvironment [10].
In a study of Lee and colleagues the neural differentiation potential of DPSCs was compared to that of stem cells from apical papilla (SCAP) , and periodontal ligament stem cells (PDLSCs) [13]. After induction in neurogenic medium for 24 h, the morphology of DPSCs, SCAP, and PDLSCs changed into neurite-like cells, including cell processes. Most of the cells were similar in shape to neuronal cells, with very thin and long cytoplasmic processes, resembling axons and dendrites. The neurogenic potential of DPSCs, SCAP, and PDLSCs was analyzed assessing the transcription levels of βIII-tubulin, microtubule-associated protein 2 (MAP2), and GFAP. In each cell line, mRNA level of the mature neuronal marker MAP2 tended to increase after neurogenic induction. The RNA level of βIII-tubulin significantly increased within SCAP and PDLSCs in neurogenic differentiation medium but not in DPSCs. DPSCs showed a significantly increased GFAP mRNA expression under differentiation conditions, whereas SCAP expressed very little GFAP mRNA in both control and differentiation groups. Interestingly, MAP2 and βIII-tubulin mRNAs were also found in undifferentiated conditions. These results suggest that SCAP might have a neural origin and thus have a capacity for neurogenic differentiation. In terms of protein level, MAP2 was strongly expressed in SCAP, DPSCs, and PDLSCs after neurogenic induction. The protein expression of βIII-tubulin increased in DPSCs under differentiation conditions, whereas GFAP was not detected. However, the expressions of neuronal markers in DPSCs were lower than those in SCAP and PDLSCs. These results indicate that PDLSCs and SCAP, as well as DPSCs, may be appropriate cell sources for neuronal regeneration [13].
Altogether these in vitro studies support the finding that DSCs are capable of differentiating into neuronal cells when cultured under appropriate inductive conditions basing on analyses of cellular morphology and expression of early neuronal markers. However, functional neurons express voltage-gated potassium, sodium, and calcium channels, which are required for the generation and propagation of action potentials [14]. The protocol of Arthur and co-workers demonstrated that DPSCs can be differentiated into neuronal-like cells by means of an inductive medium supplemented with FGF and EGF . Their protocol, however, resulted in an incomplete neuronal differentiation, since only voltage gated sodium channels could be detected without the presence of voltage gated potassium channels which are also regarded as a basic criterion for functional neuronal cell identification [4]. In a later work of Király and colleagues, DPSCs were differentiated into neuronal cells that not only express neuronal markers, but also display simultaneous voltage dependent sodium and potassium channels [15]. Their protocol consists of three steps in 10 days: pretreatment, induction, and maturation. First, DPSCs were seeded onto poly-l-lysine coated surfaces and pretreated with a medium consisting of basic FGF and 5-azacytidine. The 5-azacytidine causes hemi-demethylation of DNA leading to dedifferentiation of partly committed cells to a multipotent state. It was also reported to be a potent maturation inducing factor for neurogenesis [16]. Then, neural induction was performed by basic FGF, nerve growth factor (NGF), and neurotrophin-3 (NT-3), and the simultaneous activation of protein kinase C (PKC) and cyclic adenosine monophosphate (cAMP) pathways. In cell lines of diverse origins, it was demonstrated that neuron and glia differentiation can be promoted by activation of PKC and cAMP pathways [17]. Finally, maturation of the induced cells was achieved by continuous treatment with NT-3, and other neuroprotective factors [15]. The DPSC-derived neuronal cells generated following the protocol of Király et al. gave good results even after the engraftment into rat brain. The DPSC-derived neuronal cells were integrated into the host brain, even into the injured cortex, and showed neuronal properties not only by expressing neuron-specific markers but also by exhibiting voltage dependent sodium and potassium channels [18]. However, Király and co-workers did not observe action potential firing by the differentiated cells, addressing the incomplete differentiation of DPSCs to neuronal cells. Recently, Gervois and colleagues have implemented a two-step protocol to improve the differentiation outcome [19]. First, they adopted the neuronal induction step of Arthur’s protocol based on FGF and EGF signaling [4]. These signaling molecules are essential to induce the formation of the three-dimensional neurosphere structures, in which neural progenitor cells are close in a favorable microenvironment for the creation of close physical contacts essential for neuronal commitment [20, 21]. The second step concerning the neuronal maturation is based on activation of cAMP and NT-3 signaling pathways, as previously reported by Király [15]. Elevated intracellular cAMP is essential in sustaining neurogenic differentiation of early neuronal committed cells [22], whereas NT-3 signaling is essential for neurogenic maturation [23]. After neuronal differentiation, patch-clamp analysis demonstrated the functional activity of differentiated DPSCs by the presence of voltage-gated sodium and potassium channels selectively blocked by tetrodotoxin and tetraethylammonium, respectively. Moreover, a subset of neuronal-differentiated DPSCs was able to fire a single action potential. However, a train of repeated action potential firing after stimulation was not observed, which would be the ultimate proof of functional neurons. The failure to fire repeated action potentials might be attributed to the gating kinetics of the delayed rectifier potassium channels, that is resulting in an incomplete repolarization. The incomplete repolarization failed to deactivate sodium channels, which would be necessary for repetitive firing [19].
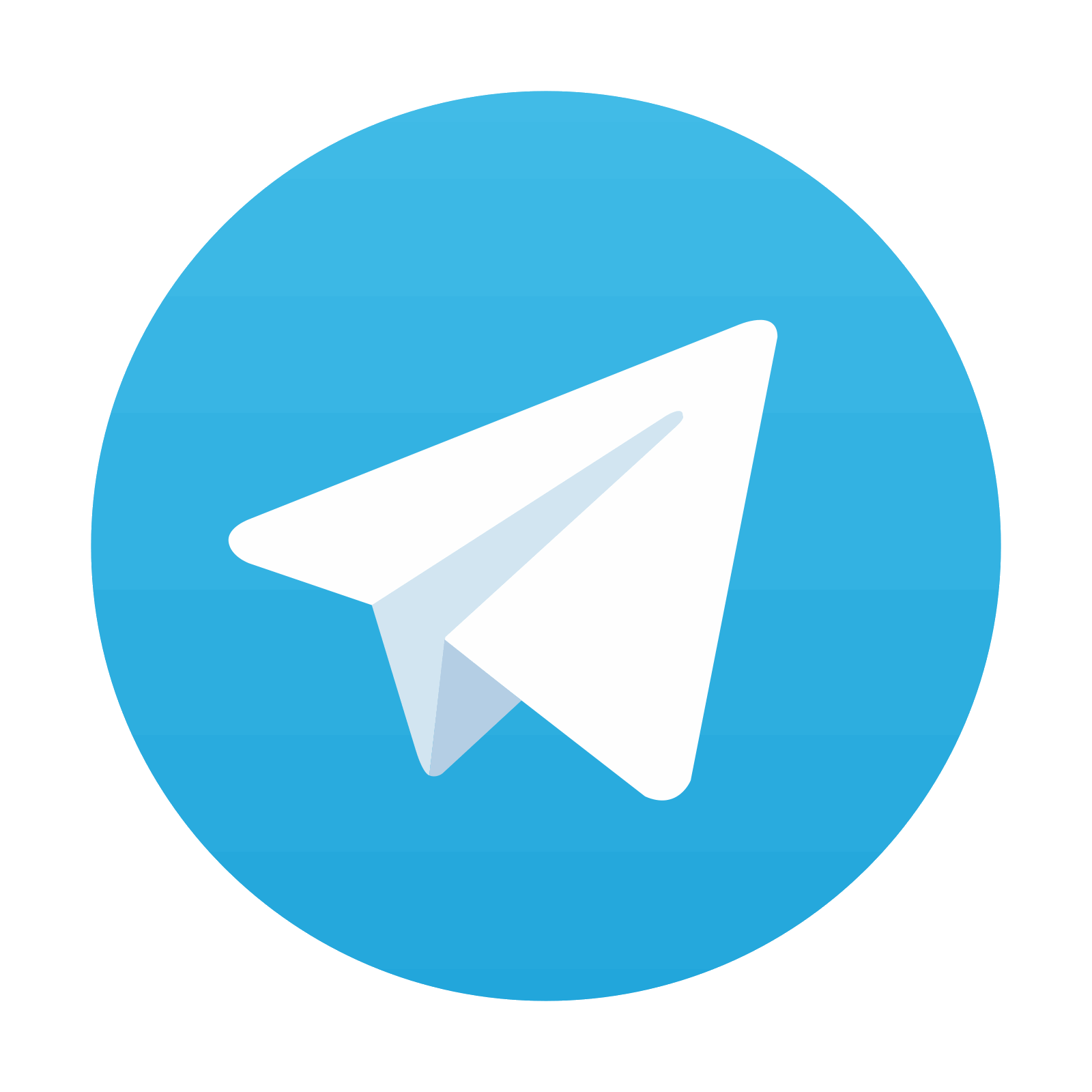
Stay updated, free dental videos. Join our Telegram channel

VIDEdental - Online dental courses
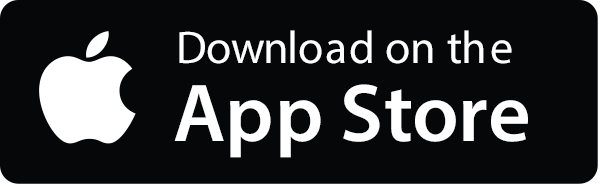
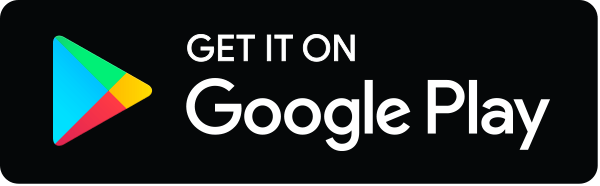