Highlights
- •
Conventional, milled, and additive manufactured polymers showed comparable wear
- •
Enamel antagonist wear was not influenced by the investigated materials
- •
Additive manufactured polymers showed decreased mechanical properties
Abstract
Objectives
To investigate the wear resistance of polymers for injection molding, subtractive and additive manufacturing of occlusal devices in comparison with enamel antagonist wear and material properties (i.e., hardness, flexural strength, and flexural modulus).
Methods
Injection molding was compared with milling and the additive technologies stereolithography, low force stereolithography, and digital light processing. For each material, eight specimens were produced for wear measurements. Extracted human premolars served as indenters. All samples were subjected to two series of a 2-body wear test consisting of 200,000 circular loading cycles with an applied load of 1) 20 N and 2) 50 N in a thermocycling environment (5/55 °C, 30 s, 3860 cycles, H 2 O). Wear resistance was characterized by means of maximum depth and volume of the resulting traces. In addition, enamel wear of the indenters and Vickers hardness, flexural strength, and flexural modulus of the polymers were determined. Wear was statistically analyzed with linear general models for repeated measures and material properties with one-way ANOVA with post-hoc Tukey-HSD tests.
Results
Wear of the antagonists was not influenced by the material (P ≥ 0.343). Likewise, no differences in wear resistance were found between materials after cyclic loading with 20 N or 50 N (P ≥ 0.074). Material properties investigated revealed decreased values for the resins for the additive manufacturing with the exception of flexural strength of one material.
Significance
Within the limitations of this in-vitro study, arylates for conventional, subtractive, and additive manufacturing of occlusal devices differ in material properties but not in wear resistance and antagonist wear.
1
Introduction
Occlusal devices are made of hard or soft polymer materials and are used in dentistry to protect teeth from excessive wear in patients with bruxism and to relieve the symptoms of temporomandibular disorders [ ]. They can be manufactured either conventionally by vacuum forming or powder-liquid mixtures of polymethylmethacrylates (PMMA) or alternatively in a digital workflow [ , ]. This includes direct digital impression taking with intraoral scanning devices or digitization of plaster models after conventional impression making, followed by computer-aided design and computer-aided manufacturing (CAD/CAM) of the occlusal device [ ]. The digital workflow is more time-efficient than traditional procedures [ ] and offers the opportunity to reproduce the occlusal devices based on the existing data set.
In case of digital manufacturing, a distinction can be made between subtractive milling and additive manufacturing using 3D printers. In the first case, occlusal devices are milled out of pre-polymerized blanks. Deviations caused by polymerization shrinkage can therefore be avoided [ ]. A major disadvantage of this technique is the waste of roughly 70% of the material, since only 1–2 splints can be milled from one blank [ ]. On the other hand, additive manufacturing comprises a variety of different technologies, with stereolithography (SLA) [ ] and digital light processing (DLP) [ ] being the most common in dentistry for processing polymers. With both of these technologies, a liquid light-curing resin is selectively cured in a vat, creating an object layer by layer. While SLA uses a UV-laser beam for point-by-point curing, DLP cures an entire layer simultaneously by projecting a light mask onto the vat. A further development of SLA is Low Force Stereolithography (LFS), where shear forces within the object during printing can be reduced by using flexible tanks. All mentioned technologies require post-processing of the printed parts, including cleaning with isopropanol and UV-curing. This is essential to enhance biocompatibility, but the degree of polymerization remains still lower compared with conventional and subtractive methods [ ].
In addition to high accuracy, occlusal devices require sufficient fracture resistance to withstand masticatory forces of 500–800 N in patients with bruxism [ ]. They should be wear resistant and also prevent the antagonists from being abraded during grinding and clenching. While mechanical properties of utilized polymers have been well investigated [ , , ], there have been only two studies on the wear resistance of CAD/CAM manufactured occlusal devices [ , ]. One study compared the 2-body wear of DLP printed occlusal devices with milled and conventional ones after 5,000 cylces with a load of 5 N [ ], the other after 120,000 cycles with a load of 50 N [ ]. Based on these studies, additively manufactured occlusal devices can be recommended for short-term use only. Therefore, the aim of the present investigation was to compare conventional, subtractive, SLA, DLP, and advanced LFS manufacturing and more recent materials in order to re-evaluate this statement and to analyze the wear of enamel antagonists. This has not been investigated before. In addition, the mechanical properties surface hardness, flexural strength and flexural modulus of elasticity of the polymers were determined. The null hypothesis assumed no differences between the evaluated materials regarding 2-body wear and aforementioned mechanical properties.
2
Materials and methods
2.1
Wear
2.1.1
Fabrication of specimens
For the wear investigation, cylindrical samples with a diameter of 32 mm and a height of 5.5 mm were designed with a CAD software (Fusion 360, Mill Vallay, California, USA). Eight samples were produced by each manufacturing method. The material compositions are presented in Table 1 .
Technique | Product name | Composition | Percentage |
---|---|---|---|
Injection molding | PalaXpress | Powder: | |
Methacrylate copolymers | > 95% | ||
1-benzyl-5-phenylbarbituric acid | < 5% | ||
Liquid: | |||
Methacrylate monomer | > 90% | ||
1,4-butanediol dimethacrylate | 5–10% | ||
Trioctylmethylammoniumchlorid | < 1% | ||
Subtractive | ProArt CAD splint | Polymethylmethacrylate | > 99% |
SLA | Dental LT Clear | Methacrylic oligomer | > 70% |
Glycol methacrylate | < 20% | ||
Pentamethyl-piperidyl sebacate | < 5% | ||
Phosphine oxide | < 2.5% | ||
LFS | Dental LT Clear V2 | Bisphenol A dimethacrylate | 50–70% |
Methacrylate monomer | 7–10% | ||
Photoinitiator | < 2% | ||
Urethane dimethacrylate | 25–45% | ||
DLP | V-Print splint | Polyesterdimethacrylate | 50–100% |
BIS-EMA | 25–50% | ||
Triethylene glycol dimethacrylate | 5–10% | ||
Hydroxypropyl methacrylate | 5–10% | ||
Diphenyl(2,4,6-trimethylbenzoyl)phosphine oxidetrimethylbenzoyl)phosphinoxid | 5–10% | ||
Butylated hydroxytoluene | <2.5% |
Subtractive manufacturing was performed by computer-numeric controlled milling (K5, vhf, software version DentalCAM 7, Ammerbuch, Germany) of transparent PMMA blanks (ProArt CAD Splint clear, Ivoclar Vivadent, Schaan, Liechtenstein). Additive manufacturing was carried out with a SLA printer (Form 2, Formlabs, Somerville, Massachusetts, USA) and Dental LT Clear resin (Vertex, Reutlingen, Germany), a LFS printer (Form 3, Formlabs) and Dental LT Clear V2 (Formlabs) as well as a DLP printer (SolFlex 170, Voco, Cuxhaven, Germany) and V-print splint resin (Voco). All samples were oriented horizontally for printing in the slicing software (PreForm 1.4.10, Formlabs; Pyramis, Voco). After printing, the post-processing protocols specified by the manufacturer were followed for each material. For Dental Clear LT, rinsing was performed for 20 min in a 99% IPA isopropanol ultrasonic bath, air drying for 30 min and 20 min UV curing (405 nm light at 80 °C) (FormCure, Formlabs). Dental LT Clear V2 corresponds to those instructions except for UV-curing performed for 60 min at 60 °C. V-Print splint samples were pre-cleaned for 2 min in a 99% isopropanol ultrasonic bath, followed by 2 min in a fresh 99% IPA ultrasonic bath. Afterwards drying for 15 min and UV-curing with flash-ligth (2000 flashes, 10 per second, 300–700 nm), cooling of 2 min and a second sequence of flash-ligth polymerisation (Otoflash G171, NK-Optik GmbH, Baierbrunn, Germany). For group injection molding, silicone molds for duplication purposes were created and embedded in cuvettes. Subsequently, the samples were produced with transparent powder-liquid mixture in a ratio of 2:1 from PalaXpress (Kulzer, Hanau, Germany) by injection molding (4 bar, 5 min; Palajet, Kulzer). The final polymerization of the resin was achieved within 30 min at a pressure of 2 bar at 55 °C in a water bath.
Afterwards, all samples were polished in a parallel grinder (Exakt 400 CS, Exakt, Norderstedt, Germany). The bottom side was polished with a standard metallographic grinding paper with grain size of 30 μm (P500). The top surface was polished in a sequence consisting of 30 μm grit (P500), 15 μm grit (P1,200) and 8.5 μm grit (P2,500) discs, respectively. Prior to the experiment, all samples were stored in a water bath for 24 h at 37 ± 1 °C and subsequently fixed in the sample holder of the chewing simulator with an autopolymerizing resin (Protemp, 3 M, Saint Paul, Minnesota, USA).
2.1.2
Fabrication of enamel indenters
As indenter, eight human premolars without fillings or carious lesions were selected from a population of anonymously donated extracted teeth. These were stored in 0.5% chloramine solution at room temperature and avoiding light exposure after extraction. After removal of the roots and the pulp, the anatomical crowns were fixed to a metallic sample holder with PatternResin (GC, Tokyo, Japan) with the tip of the buccal cusp perpendicular to the surface of the specimen when in contact. The crowns were polished with a goat hair brush (Henry Schein, Langen, Germany) and marks were placed on their vestibular side to allow matching of teeth and specimens, and to ensure a constant orientation in the chewing simulator. Prior to loading all teeth were digitized with a lab scanner (D2000, 3shape, Copenhagen, Denmark).
2.1.3
2-body wear test
Eight samples of each material were mounted in a computer-controlled dual-axis chewing simulator (Type CS-4.8, SD Mechatronik, Feldkirchen-Westerham, Germany) and loaded with the enamel indenters for 200,000 cycles and a load of 20 N each. A circular movement with a diameter of 3 mm was performed at a potential penetration depth of 1 mm corresponding to 2.8 mm of contact trace ( Fig. 1 ). Setting a circulation speed of 20 mm/s, this resulted in a dynamic loading frequency of 2.1 Hz. During loading, thermocycling was carried out at 5 °C and 55 °C for 30 s dwell time and 15 s change time. The integrated force and wear measuring system (PM-3, SDM) allows the separate evaluation of two-dimensional specimen and indenter wear in the vertical axis in real-time by using piezo distance measurement sensors mounted to both the upper cross-beam and the indenter-mount. The specimen wear was recorded every 500 cycles. For monitoring the enamel antagonist wear, the indenter moved to an unalterable metallic reference platform until contact every 5,000 cycles to determine wear in the vertical axis. Specimen wear monitoring was automatically corrected for indenter wear.

After the 200,000 cycles were completed, the indenters were removed and digitized with the lab scanner D2000. Reposition according to the previous orientation was based on the marks, and the specimens of the next material were loaded. The experiment was repeated with a load of 50 N for 200,000 cycles on a second trace using the same specimens. In total, the enamel antagonists were loaded for 2 × 10 6 cycles and digitized 10 times each.
2.1.4
Enamel indenter wear measurement
The wear was analyzed on the basis of the scans. These were imported as standard tessellation language format into an inspection software (Geomagic Control X, 3D Systems, Rock Hill, South Carolina, USA) where the subsequent scans were superimposed based on an initial fit followed by a best-fit according to Gauss. The cusp tips as a region of wear were excluded from this superimposition procedure. Finally, a three-dimensional surface comparison was carried out to determine the maximum deviation in the area of the cusp tips after every 200,000 cycles.
2.1.5
Specimen wear measurement
The specimens were analyzed with a 3D optical profilometer at x40 magnification (VR-5200, Keyence, Osaka, Japan). The maximum vertical intrusion depth and the volume loss were determined separately for the traces after loading with 20 N and 50 N and topographical profiles were applied to illustrate the wear.
2.2
Vickers hardness measurements
The hardness of all samples (N = 40) was tested after 24 h water storage at 37 ± 1 °C. One measurement per sample was performed according to the Vickers method (Q10 A, software Qpix T2, Qnee, Mammelzen, Germany). For this purpose, the plane grinded specimens were vertically loaded with an indenter in the form of a diamond pyramid and cone angle of 136° and afterwards the penetration depth was measured. The dwell time was 10 s and the test load 2 N. Subsequently, the diagonals of the indentation were measured at x20 magnification and the mean value of the indentation diagonals was determined to calculate the Vickers hardness (HV) using the following formula:
HV=0.18914Fd2
F is the test load in newton.
d is the mean value of the penetration diagonals in mm.
2.3
Three-point bending test
The three-point bending test was carried out in accordance with ISO 20795-1:2013 – Dentistry-Base polymers. First, five specimen strips with a length of 64 mm × 10.2 mm × 3.7 mm of each material were fabricated. The production was carried out in the same way as the specimen for wear measurement. The width was then grinded to 10 ± 0.05 mm with the parallel grinder Exakt 400 CS and metallographic grinding paper (P500). The height was electronically controlled grinded to a thickness of 3.3 mm in three steps (P500, P1.000, P1.200). An electronic caliper (accuracy of ± 0.01 mm) was used to verify the height (3.3 ± 0.05 mm) and width (10 ± 0.05 mm) at three points each. All samples were then stored in distilled water for 50 ± 2 h at a temperature of 37 ± 1 °C. In contrast to ISO 20795-1:2013, the samples were taken from the water bath immediately before testing and a three-point bending test was carried out (Zwick 010, software testXpert II, ZwickRoell, Ulm, Germany) with a constant displacement rate of 5 mm/min until fracture ( Fig. 2 ). The maximum flexural strength, σ, in megapascal (MPa) was calculated with the following formula:
σ=3Fl2wh2
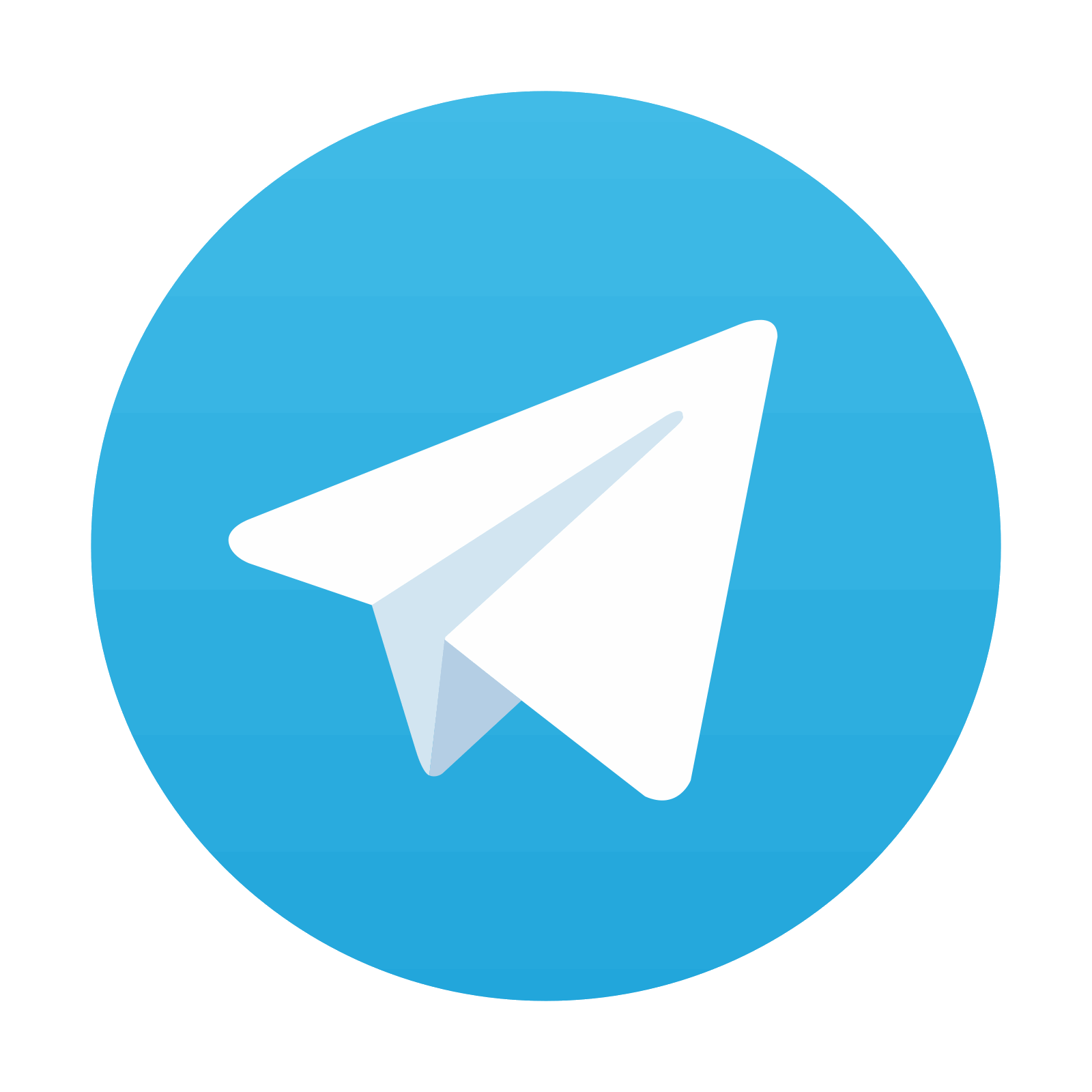
Stay updated, free dental videos. Join our Telegram channel

VIDEdental - Online dental courses
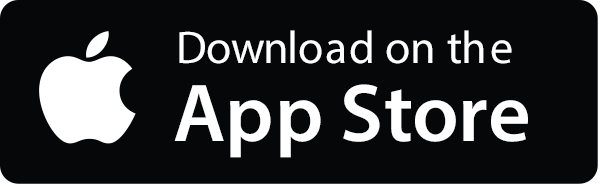
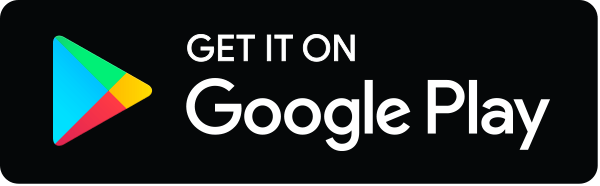
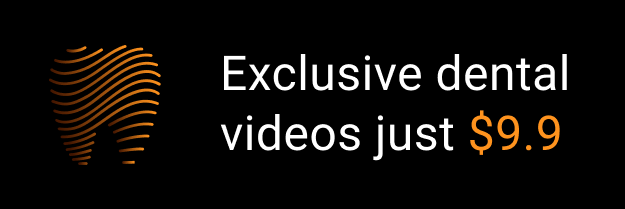