Fig. 1
Didactic classification of epigenetic modifications (reproduced with permission from [10]). (a) DNA methylation schematic illustrating the repeating nucleosome, which a single strand of DNA wound round the histone core. Methyl groups are covalently attached in CpG islands. (b) Histone modifications: the nucleosome comprises eight core histones. Linker DNA, connects the nucleosome structures and an additional histone protein (H1), enables further DNA compaction. The nucleosome core has a relatively long N-terminal amino acid extension, which is subject to several covalent epigenetic modifications (methylation, acetylation, phosphorylation, ubiquitination and SUMOylation). Histone modifications control both the conformation of the chromatin structure leading to an open or closed chromatin state and also nucleosome positioning. (c) The varying architecture of chromatin and position of the nucleosome regulates transcription, with an open conformation being transcriptionally active and a closed structure being transcriptionally repressive
Pharmacological agents, which epigenetically modify histone acetylation, include histone deacetylase inhibitors (HDACis) . These molecules can reversibly change gene expression resulting in altered SC fate [18, 19]. Several HDACis, including Trichostatin A (TSA), Valproic acid (VPA) and Butyric acid, can induce SC proliferation, differentiation and also have anti-inflammatory activity [18, 20–22]. From a dental perspective, HDACis have been shown to epigenetically-modulate postnatal dental pulp SC (DPSC) differentiation and self-renewal potential due to their ability to alter the balance between histone deacetylases (HDACs) and histone acetyl transferases (HATs) [23]. Application of HDACis prevents deacetylation of the N-terminal histone tail within the nucleosome, leading to changes in chromatin architecture and transcription activity [24]. Eighteen human HDAC enzymes have been identified which differ in their expression profiles and cellular localisations [25]. While the entire functional role of HDACs is still to be fully elucidated [26], pan-HDACis and isoform specific inhibitors have been developed. Notably application of pan-HDACi have been shown to accelerate differentiation and mineralisation events in dental pulp cell populations [18, 27], although de-differentiation induction has also been shown in some immature SC populations [28].
In endodontics , tissue-resident and local SC populations including DPSCs and Stem Cells from the Apical Papilla (SCAP) may be key to regenerative responses [4]. These SCs are relatively easily accessible and have therapeutic application at other oral and bodily locations. For repair processes SC migration, proliferation and differentiation are necessary [29] and reportedly these cellular regenerative-associated events are epigenetically regulated with the role of HDACis in modifying these processes being recently highlighted [30, 31]. ‘Regenerative endodontics’ utilising SC technology continues to gather significant momentum and involves concerted academic and clinical effort to develop novel tissue engineering approaches which aim to utilise cell-free homing, platelet–derived plasma and triple antibiotic paste [32–35]. Targeted restorative dental materials, which harness innate biological reparative processes utilising epigenetic therapeutics may also have clinical utility in the more immediate future [36].
Furthermore for clinical application of dental SC technologies a detailed working knowledge of their cell isolation, expansion, and biobanking combined with knowledge of genotoxicity will be essential for clinical application. Training of the clinical team in these areas will be important to realising patient benefit with this technology. The aim of this chapter therefore is to review current knowledge of dental SCs and how epigenetics may play a role in their responses during health, disease and dental clinical application.
Review
Dental Stem Cells
Postnatal SCs, in particular ones derived from dental sites, provide a readily available source for tissue engineering and repair strategies throughout the body [2]. DPSCs and stem cells from exfoliated deciduous teeth (SHED) , reportedly account for 1–5 % of total permanent and 2–9 % deciduous pulp cell populations, respectively [4, 37, 38]. Other dentally relevant SC sources have also been identified [2] and include stem cells of the apical papilla (SCAP), dental follicle progenitor cells (DFPCs) and periodontal ligament stem cells (PDLSCs) [38–40].
DPSCs are one of the most readily available SCs as they can be isolated by the dental practitioner from extracted third molar teeth which is regarded clinically as ‘waste tissue’. Notably harvesting from this site avoids the donor site morbidity typically observed at other surgical locations. While the therapeutic application of DPSCs, such as dentine-pulp regeneration, appears quite promising, their overall utility may be limited due to the availability of autologous molar teeth for extraction along with the need for their in vitro expansion to obtain sufficient numbers for use [41]. Indeed during culture expansion, phenotypic change [42] may occur which also limits their clinical application. An understanding of the mediators, which are often epigenetic in nature, controlling self-renewal and lineage commitment, is therefore important. For regenerative dentistry other dental SC sources, such as DFPC and PDLSC may either be limited in their application due to diminished differentiation potential or in their availability [2, 8, 38]. SHED, however, are considered a rich source of dental SCs which can be harvested from waste tissue from extracted or exfoliated deciduous teeth. These dental SCs can be commercially biobanked as intact pulp tissue and subsequently SHED are isolated and expanded in vitro following enzymatic digestion of pulp tissue combined with fluorescence-activated cell sorting [43]. Although SHED are relatively easily obtained [43], currently their routine harvesting and clinical application is reportedly hampered by infection, lack of viable pulp tissue as well as lack of a standardised medicinal good manufacturing practice [37, 38].
Epigenetic Modifications and Dental Stem Cells
Classically ESCs exhibit an open chromatin structure characterised by relatively low levels of DNA methylation and high levels of histone acetylation [44]. After cellular lineage commitment and differentiation, the chromatin changes to a more tightly-bound conformation with reducing levels of acetylation and increasing levels of DNA methylation [45]. It is now evident that ESCs are maintained in a self-renewing state by a complex network of transcription factors [46], while differentiation and developmental potential are under epigenetic control [47]. During differentiation the pluripotency genes, Oct4 and Nanog, are repressed [48], an effect which is mediated initially by histone modifications, with the downregulation maintained and cell reprogramming prevented by DNA methylation processes [49].
DNA methylation is cell type dependent and was traditionally thought to occur exclusively at CpG islands (Fig. 1), however, recently it was noted that up to 25 % of all ESC methylation was in a non-CG context [50]. Indeed the observed pattern of non-CG methylation disappeared when differentiation processes were induced in ESCs supporting a crucial role for methylation in determining cell fate [50]. Further characterization of epigenetic modifications in ESCs identified hypermethylation at 3 % of promoter regions in CgG islands, and notably the regions included developmental genes such as Rhox2 [51]. It has been suggested that although global methylation levels of CpG may be similar between cell types the distributions of methylation marks in ESCs are unlike any other cell type [52]. Other epigenetic modifications are also important regulators of SC function and self-renewal with HDACs being vital to maintaining the self-renewal capabilities of mesenchymal SCs [7, 53] by maintaining the expression of key pluripotent transcription factors [6]. Amongst the best characterised cellular mediators of acetylation and methylation processes in SCs are the polycomb and trithorax protein complexes, which occupy ESC gene promoter sites and assist in catalysing specific histone modifications [54, 55]. Over the last 10 years knowledge of non-coding (nc) RNA, such as microRNA, has developed in relation to their epigenetic control due to their dysregulation in diseases such as cancer [56], their regulation of epigenetic mechanisms such as DNA methylation [57] and conversely their expression is regulated by epigenetic modifications [58]. Other ncRNAs, such as long-ncRNAs, also epigenetically modulate chromatin architecture by recruiting and binding chromatin-modifying proteins to specific genomic sites [59], while also guiding proteins, such as histone methyltransferases to those sites [60, 61].
Recently, interest in the epigenetic control of DPSC behaviour has led to suggestions that dental developmental anomalies, such as dentine dysplasia and dentinogenesis imperfecta, may be related to epigenetic modifications during odontoblast differentiation [62]. However, current research in this area is preliminary and has not, to date, analysed pure SC populations [63]. In a recent SC study, epigenetic states and related differentiation profiles were analysed in DPSCs and DFPCs via their expression of odontogenic genes, such as dentine sialophosphoprotein (DSPP) and dentine matrix protein-1 (DMP-1). Transcript levels were epigenetically-suppressed in DPFCs, while osteogenic stimulation in vitro demonstrated significant mineralisation increases only in DPSCs [8]. Interestingly, a highly dynamic histone modification response was demonstrated in mineralising DPFCs, but not in DPSCs, and the latter cells also expressed relatively high levels of the pluripotency-associated transcripts, Oct4 and Nanog. It was concluded that these two neural crest-derived SC populations were distinguished by epigenetic repression of dentinogenic genes with dynamic histone enrichment in DPFCs during mineralisation. These data demonstrated the essential role of epigenetic mechanisms in DPSC terminal differentiation and in controlling cell phenotype and lineage commitment [8].
Histone Acetylation Related Stem Cell Effects
Histone acetylation controls cellular transcription by altering the conformation of chromatin, a process balanced homeostatically by two cellular enzymes histone deacetylases (HDACs) and histone acetyl transferases (HATs) . HDACs in particular have been a focus of attention as potential therapeutic targets [21], with 18 human HDAC family members characterised and divided into four classes [64]. Class I members (HDAC-1, -2, -3, -8) are ubiquitously expressed predominately in the cell nucleus [25], while the expression of class II enzymes (HDAC-4, -5, -6, -7, -9, -10) are tissue-restricted and dynamically shuttle from nucleus to cytoplasm [21, 65]. A recent analysis of selected HDAC expression in extracted third molar teeth demonstrated that HDACs -2 and -9 were expressed in selected pulp cell populations, while HDACs -1, -3 and -4 appeared only relatively weakly expressed [66]. The reported variations in tissue expression suggest different cellular roles for HDACs and indications are that class I HDACs regulate cell cycle progression and maintain ESC pluripotency, while class II HDACs are central to stem cell differentiation and mineralisation processes [6, 67, 68]. However, it is likely that their roles inter-link, with both Class I and II HDAC enzymes playing crucial roles in maintaining the pluripotency of stem cells and in governing cell fate [6, 53]. The labile effects of histone acetylation can be therapeutically targeted by HDACis, which alter the homeostatic HAT/HDAC balance, leading to changes in transcription and subsequently, stimulating pleiotropic cellular effects involving growth, differentiation and regeneration [31, 69, 70].
HDACi-Induced Stem Cell Effects
Several HDACi including TSA, VPA and SAHA, have been used already in clinical cancer trials, and their promise in treating neurodegenerative. conditions, as well as in bone engineering and regenerative medicine has also been highlighted [16, 71, 72]. Indeed, several HDACi, at relatively low concentrations, have been demonstrated to induce differentiation and increase mineralisation dose-dependently in primary dental pulp cell (DPC) populations containing stem cells [18, 23, 27]. Furthermore, HDACis and VPA in particular has also been shown to improve both the efficiency of somatic cell reprogramming to iPSCs, as well as increasing the expansion capacity of selected ASC populations [19, 73].
Certain HDAC members, -1 and -2, have been identified as playing crucial roles in controlling cellular proliferation and stem cell self-renewal by maintaining the expression of key pluripotent transcription factors such as Oct4, Nanog, Esrrb, and Rex1 [6]. In vitro HDACi stimulate the expansion of hematopoietic SCs, while decreasing the relative number of differentiated cells [19, 74], whereas in vivo HDACi-induced an increase in SC markers following digit amputation in mice [75] and during kidney organogenesis in zebrafish [30]. Conversely, several studies using various cell types have demonstrated that HDACi can reduce stem cell proliferation and promote terminal differentiation [76, 77, 78, 53]. These apparently divergent effects of HDACi were attributed to the level of baseline cell differentiation with stem cells tending to de-differentiate, while precursor blast cells differentiated [74]. Other researchers have further speculated that in addition to cell status, HDACi-dose may all be critical to the cell response, with undifferentiated cells and high doses of HDACi tending to promote differentiation, while low doses applied to cells undergoing self-renewal are more likely to stimulate a de-differentiation effect [28, 79].
Within dentistry several recent in vitro studies using mixed primary cell cultures [27], and pure DPSC populations [18, 23] have demonstrated an HDACi-induced stimulation of odontoblast-like cell differentiation with an associated increase in matrix mineral deposition. The effect of epigenetic modulation on regenerative processes highlighted differences between normal and immortalised cells with lower concentrations of HDACi and shorter durations of exposure being required to promote reparative effects in primary cells [27]. The effect of HDACis on human DPSC populations in vitro demonstrated increases in mineralisation, associated mineralisation-marker gene expression and down-regulation of HDAC-3 [18] and HDAC-2 [23], respectively. In vivo the response to a pre-natal systemic injection of TSA in dentine-pulp development was studied by histological analysis of tooth development at 7-days post-natal [23]. The volume of dentine deposited was thicker (1.64-fold) and the number of odontoblasts in the measured area was higher (1.74-fold) in the TSA group compared with the control [18]; this was attributed to an accelerated HDACi-induced differentiation effect. This reported ability of HDACi to manipulate stem cell fate renewal, although complex, is interesting from a regenerative medicine perspective as it potentially enables the scientist and clinician to promote differentiation, for example in tissue engineering, or electively maintain pluripotency if expanding SCs for cell-based therapies.
Induced Pluripotent Stem Cells (iPSCs)
The translational potential of pluripotent ESCs use has been somewhat limited due to expense, storage issues, ethics, as well as issues relating to immunological rejection [80]. In an attempt to address these issues, patient’s own somatic cells have been reprogrammed into induced pluripotent stem cells (iPSCs) using a combination of four transcription factors (Oct4, Sox2, Klf4, c-Myc) and a viral induction protocol [81, 82]. However, as safety concerns were raised over the use of the proto-oncogenes, Klf4 and c-Myc, and low reprogramming rates (<0.001 %), the epigenetic modifiers, HDAC and DNA methyltransferase inhibitors, were used with and without these transcription factors [73]. The use of HDACi, in particular VPA, combined with all four transcription factors improved reprogramming efficiency up to 100-fold [73]. The presence of VPA, but absence of c-Myc, also increased reprogramming efficiency levels; however, when klf4 was eliminated the efficiency was greatly reduced indicating the importance of this molecule in the process [83]. It subsequently appears that VPA acts by inducing Oct4 predominately through the PI3K/Akt/mTOR pathway [84]. Further optimisation of iPSC techniques have been developed focusing on viral-free methods and avoidance of genomic modification approaches by using synthetic modified mRNAs for Oct4, Sox2, Klf4, and c-Myc, [85]. Although, VPA supplementation has also been combined with the synthetic mRNA methodology, only minor increases in reprogramming efficiency were observed [85]. The role of HDACi-supplementation in reprogramming demonstrates the pivotal role of epigenetic modification in the de-differentiation process and indicates the importance of the open chromatin architecture characteristic of ESCs [52].
Conclusions
A picture of the influence of epigenetic modifications on the mechanisms controlling stem cell fate and differentiation is developing, which critically involves DNMT and HDAC enzyme function, cell maturity and environmental signals. Further elucidation of the role of these enzymes is required to understand the influence of epigenetic modifications on pluripotency, their influence on stem cell fate and role in regenerative responses prior to any proposed clinical application. Regenerative tissue engineering approaches require SCs, signalling mediator molecules and a scaffold within which the cells can operate [86]. Therapeutically, HDAC and DNMT inhibitors could epigenetically manipulate the self-renewal capacity of SCs in vitro or indeed in vivo in the implanted stem cells. Additionally, there are opportunities for pharmacological epigenetic inhibitors to improve the efficiency of somatic cell reprogramming to iPSCs for subsequent craniofacial tissue regeneration, as availability of sufficient SCs may be limited. Finally, the availability of DSCs, their isolation, expansion, and epigenetic manipulation with HDACi could, at the very least, lead to a better understanding of DSC biology, which will enable their future clinical use within regenerative medical strategies.
References
1.
Dupont KM, Sharma K, Stevens HY, Boerckel JD, Garcia AJ, Guldberg RE (2010) Human stem cell delivery for treatment of large segmental bone defects. Proc Natl Acad Sci U S A 107:3305–3310CrossRefPubMedPubMedCentral
2.
Huang GT, Gronthos S, Shi S (2009) Mesenchymal stem cells derived from dental tissues vs. those from other sources: their biology and role in regenerative medicine. J Dent Res 9:792–806CrossRef
3.
4.
Gronthos S, Mankani M, Brahim J, Robey PG, Shi S (2000) Postnatal human dental pulp cells (DPSCs) in vitro and in vivo. Proc Natl Acad Sci U S A 97:13625–13630CrossRefPubMedPubMedCentral
5.
Ware CB, Wang L, Mecham BH et al (2009) Histone deacetylase inhibition elicits an evolutionarily conserved self-renewal program in embryonic stem cells. Cell Stem Cell 4:359–369CrossRefPubMedPubMedCentral
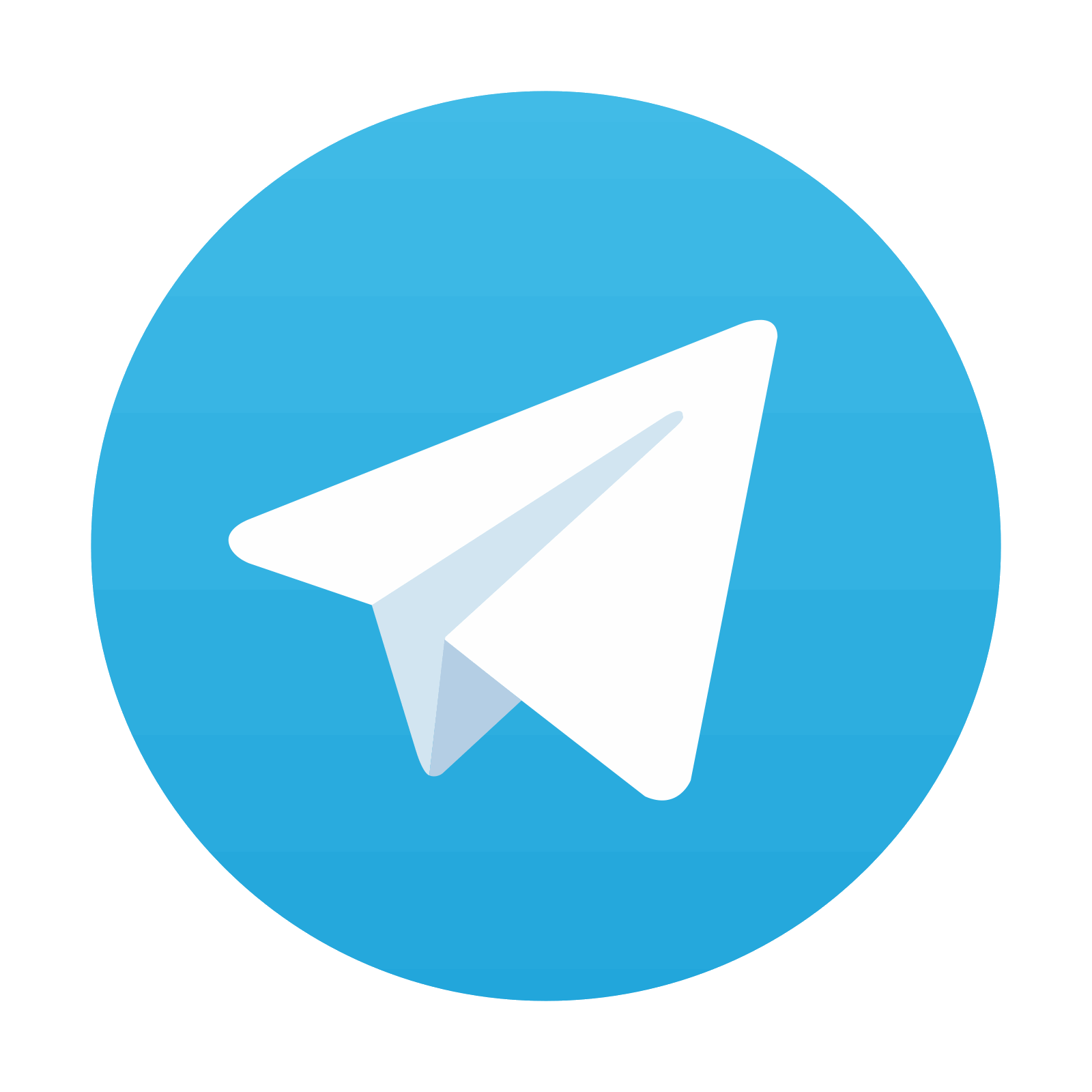
Stay updated, free dental videos. Join our Telegram channel

VIDEdental - Online dental courses
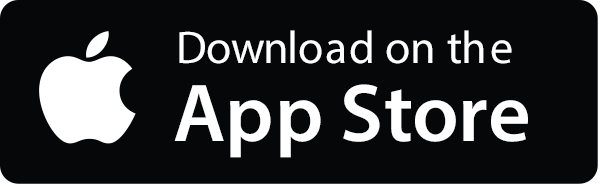
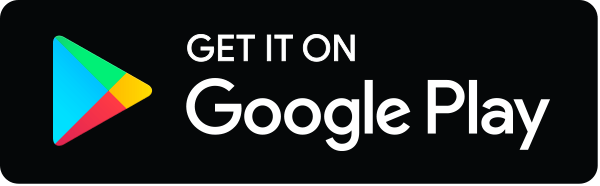