Fig. 7.1
Causes of structural failures in root-filled teeth [6]
Dentin is a biological composite material consisting of inorganic phase (50 vol%), organic phase (30 vol%) and water (20 vol%) [20]. The loss of dentin and other age-, bacterial- and chemical-mediated changes to dentin matrix would compromise the mechanical integrity of root dentin and demands attention. In this line, optimization of dentin characteristics such as ultra-structural integrity, mainly from a mechanical/chemical perspective, in order to improve the mechanical properties and resistance to enzymatic degradation would facilitate the successful management of previously infected dentin tissue. Currently novel nanotechnology-based treatment approaches that produce significant antibiofilm efficacy and at the same time enhance the ultrastructural integrity of dentin tissue are being developed for the management of infected dentin during root canal treatment and minimally invasive dental caries management. This chapter will present the biomaterial aspects of dentin, issues related to dentin degradation and potential treatment methods available to stabilize the dentin matrix. Various stabilization methods and their effect on specific dentin properties will also be covered.
7.2 Dentin
Dentin at a structural level exists as a fiber-reinforced composite (Fig. 7.2). The less mineralized intertubular dentin forms the matrix, and the highly mineralized peritubular dentin forms the fibre reinforcements. It is traversed by dentinal tubules that run continuously through the bulk of the dentin. Thus, dentin microstructure is basically a scaffold of organic fraction on which inorganic fraction is well distributed [20]. The hierarchical order of dentin aids in understanding its structural and mechanical properties [21].
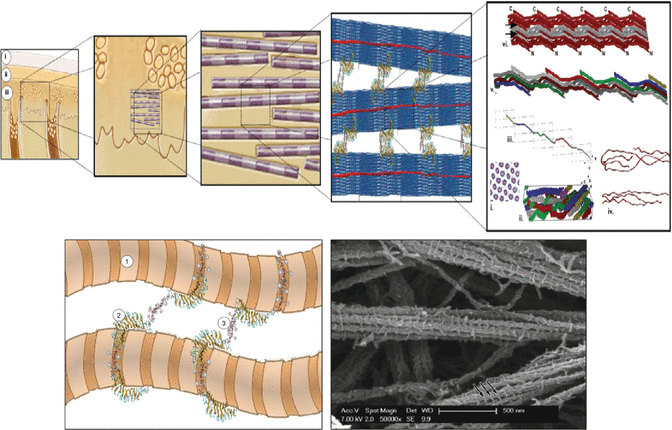
Fig. 7.2
Dentin presented in a hierarchical fashion. At structural level it exists as a fiber-reinforced composite. A not to scale schematic sketch of the interfibrillar supramolecular assemblies that interconnect collagen fibrils: (1) collagen fibril; (2) decorin protein core; (3) chondroitin 4-sulfate glycosaminoglycan. The known periodicity of these interfibrillar aggregates in register with the gap zones of collagen fibrils, present in most connective tissues, remains uncertain for mineralized tissues. (C) A high magnification image of a sample of acid-soluble collagen and decorin treated with cupromeronic blue, which reacts with glycosaminoglycans and demonstrates their assembly as interfibrillar co-aggregates (arrows) (Adapted from Bertassoni et al. [21]. With permission from Elsevier)
The inorganic component in dentin is mainly carbonated nanocrystalline apatite minerals. These minerals are closely associated with the collagen scaffold, either intrafibrillar or extrafibrillar [22]. The apatite crystals (≈5 nm thick) are needle like near the pulp and plate like towards the dentino-enamel junction. Type I collagen is the major structural protein making up to 90 % of the organic fraction, and the rest are non-collagenous proteins [20]. Type I collagen, roughly 100 nm in diameter, exists as fibrils in dentin which are stabilized by endogenous covalent intermolecular cross-linking [23]. The collagen fibrils are randomly oriented in a plane perpendicular to the plane of dentin formation or axis of dentinal tubules [24]. In addition, the three-dimensional collagen network exists in an aqueous environment.
The 10 % of the organic phase consists of various phosphoproteins and other non-collagenous proteins. Proteoglycans and glycosaminoglycans (GAGs) are known to be closely associated with collagen fibrils via hydrogen bonds. The proteoglycans and the hydrophilic anionic GAG side chains interact with one another, forming interfibrillar bridges. These molecules absorb water and span the interfibrillar spaces, thereby regulating mechanical properties of the dentin collagen matrix [21]. The structural integrity of dentin provided by the inorganic and organic fraction is crucial to retain the function of a tooth (Fig. 7.3) [12]. In a root-filled tooth, other than the loss of dentin, changes in the physical properties of dentin matrix could also contribute to the increased propensity of fractures [12, 25].
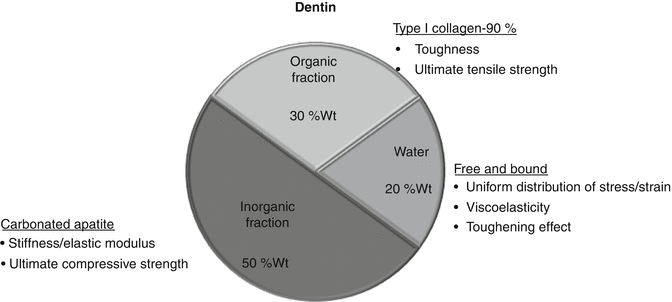
Fig. 7.3
The role of different constituents of dentin on its structural integrity
The mechanical stability of biological composites depends on the optimum balance between toughness and stiffness [26]. The mechanical properties of dentin such as Young’s modulus, strength, and fracture toughness are the result of the complex interactions of its constituents as well as the microstructural arrangement. Toughness is the total energy absorbed by a structure before it fractures (fracture resistance), whereas Young’s modulus is the ratio of strength vs. strain within the elastic limit. The collagen fraction of dentin contributes to its toughness and ultimate tensile strength, while the mineral fraction contributes to its elastic modulus and compressive strength [12, 27]. The collagen fraction also acts as a cushion in between the inorganic elements of dentin [12, 28]. In bulk dentin, the alignment of the dentinal tubules also governs its mechanical properties [24].
The water content of dentin exists as a free or ‘unbound’ water and ‘bound’ water. The unbound water is found in dentinal tubules and other porosities in the dentin [29]. This water keeps the dentin matrix bathed in minerals such as calcium and phosphate. The bound water in dentin is associated with the inorganic apatite crystals and organic phase (collagen and non-collagenous proteins). This water forms a monolayer on the surface of hydroxyapatite via hydrogen bonds and van der Waals forces [30]. Bound water also forms an integral part to stabilize the triple helix of collagen molecules. Each tripeptide is known to be stabilized by two water molecules [31]. Loss of water in dentin reduces the elastic modulus of dentin from 23.9 to 20 GPa [28]. Partial dehydration at room temperature for 7 days would result in increase in stiffness and decrease in the toughness values significantly [29].
7.3 Physical and Mechanical Alterations in Dentin Matrix
7.3.1 Non-iatrogenic Causes
Degradation of dentin matrix is a common observation in endodontically treated teeth [15]. The disease processes/bacterial proteases degrade dentin collagen. It is evident with an increase in the years of clinical functioning [15]. Bacterial biofilms degraded the dentin surface collagen to various degrees depending on the growth environment [32, 33] (Fig. 7.4). Clinically, internal resorption of root dentin can occur in up to 75 % of teeth with post-treatment disease [34]. Bacterial collagenolytic enzymes and activation of host-derived matrix metalloproteinases MMPs are some of the possible reasons for resorption/degradation [35]. This dentin surface change has been linked to interfacial failure at the biomaterial–dentin interface of root-filled teeth [36].
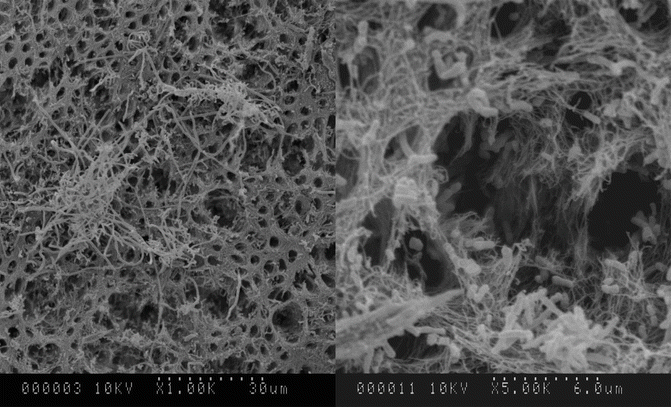
Fig. 7.4
Degradation of dentin by bacterial biofilms grown in vitro on dentin. Areas of demineralization as well as collagen degradation were evident on the surface
The degradation of the exposed dentin collagen could be due to (a) extrinsic factors such as enzymatic hydrolysis by salivary leakage and/or (b) intrinsic factors such as bacterial and host-derived MMPs [15, 37–39]. These MMPs play a vital role in degradation of exposed collagen fibrils created during dentin bonding procedures [40, 41]. Incompletely infiltrated collagen fibrils within the hybrid layers formed during dentin bonding were degraded by the host-derived proteases in the absence of bacteria [15, 42]. Commonly used acids such as EDTA and phosphoric acid are also capable of activating the host-derived protease activity [43]. Inhibitors of MMPs have been studied extensively to control the pathological degradation of ECM. Synthetic peptides, chemically modified tetracycline, bisphosphonates, natural compounds and chlorhexidine are considered as potential MMP inhibitors [43, 44].
7.3.2 Iatrogenic Causes
The iatrogenic factors usually complement the changes caused by the pathological process in dentin, which would further compromise the ultrastructural/mechanical integrity of teeth [10, 12, 13, 34, 45]. Commonly used root canal irrigants remove the inorganic phase and/or the organic phase of the dentin [12, 17]. Removal of the organic and inorganic fractions of the dentin compromises the mechanical integrity of dentin. Overzealous application of chemicals/medicaments leads to erosion and microcracking of dentin [12, 15, 40]. EDTA and sodium hypochlorite (NaOCl) are commonly used for root canal debridement/disinfection. NaOCl is a deproteinating agent that results in the heterogeneous removal of organic substrate from dentin. Use of higher concentrations of NaOCl for longer duration could produce more deleterious changes to dentin matrix as compared to the application of lower concentrations of NaOCl for shorter duration due to their greater ability to interact with collagen matrix. This interaction would reduce the flexural strength of dentin [45]. Microhardness of dentin was significantly reduced when subjected to chemicals such as NaOCl, chlorhexidine, hydrogen peroxide and EDTA [46, 47].
The changes in dentin substrate following the application of common root canal irrigants resulted in almost 75 % decrease in the mechanical strength [17]. NaOCl resulted in the decrease of mechanical strength of root dentin up to 59 % [48]. Similarly, the common intracanal medicament Ca(OH)2 also reduced the mechanical strength by 32 % [48]. The caustic and strong alkalinity of these chemicals may denature the carboxylate and phosphate groups, which leads to the collapse of dentin structure. In addition to the chemical effects, routine instrumentation and root-filling procedures involves removal of bulk dentin, which may further jeopardize the mechanical integrity of root dentin [49]. Despite many attempts to improve the resistance to fracture of root-filled teeth with the help of a filling material, till date, there is no such treatment available that restores the mechanical integrity of the remaining dentin. Although the iatrogenic and non-iatrogenic changes occur in the inner aspect of root dentin, they could act as vulnerable areas of crack initiation/stress concentration, which can further increase the predilection of vertical root fractures in teeth [12, 50].
7.4 Dentin Tissue Stabilization
Tissue stabilization is the process of rendering the ultrastructure of a tissue more stable in order to provide or enhance its mechanical properties and resistance to chemical-mediated degradation. Dentinal collagen stabilization has been explored extensively to improve the resistance to degradation in hybrid layers, to improve the bond strength in case of adhesive restorations, to manage dentinal hypersensitivity and also to stabilize surface dentin of root canal walls. All these aspects have the same objective, which is to cross-link dentin collagen in order to improve its stability. Cross-linking the dentin collagens protects them from host-derived MMPs and bacterial proteases. Cross-linking of collagen could be achieved by several approaches, which can be broadly categorized as chemical or physical approaches (Table 7.1). The cross-linking process is meant to improve the mechanical and physical characteristics of collagenous tissues and scaffolds [51–53]. The following paragraphs will discuss various collagen cross-linking methods that have been used in biomaterials with relevance to dentistry.
Table 7.1
Various methods available to obtain crosslinking of collagen
Enzyme mediated
|
Chemical agents
|
Photoactivated
|
Others
|
---|---|---|---|
Transglutaminase
|
1. Crosslinking of amino groups
|
1. Synthetic polymers
|
|
Glutaraldehyde
|
Poly (vinyl alcohol)
|
||
Glyceraldehyde
|
3. Tris(bipyridine)ruthenium(II) chloride + [8]
|
Poly (acrylic acid)
|
|
Epoxy compounds
|
Polyethylene
|
||
Genipin
|
Poly (vinyl pyrrolidone)
|
||
2. Direct crosslinkingof polypeptide chains
|
2. Hyaluronic acid
|
||
Carbodiimides(EDC/NHS/MES)
|
3. Heparin
|
||
3. Chlorhexidine
|
4. Chondroitin-6-sulfate
|
7.4.1 Chemical Cross-Linking
Chemical cross-linking is one of the most widely used approaches. Aldehydes such as formaldehyde, glutaraldehyde, epoxy compounds, etc. have been used in fixing biological tissues including dentin collagen [52, 54]. Cross-linking of collagen using glutaraldehyde increases the tensile properties and stiffness of demineralized dentin [55, 56] and increases resistance to enzymatic degradation (collagenase) [57]. Dentin collagen cross-linking has also been reported as a treatment for hypersensitivity using various resins and glutaraldehyde [58]. Glutaraldehyde treatment decreases dentin sensitivity by several mechanisms: (1) Cross-linking of dentinal collagen. (2) The amino group–containing organic fraction in dentin reacts with GD, which results in polymerization of HEMA. (3) Plasma proteins in dentinal fluid react with GD, which results in precipitation. However, one of the main disadvantages of glutaraldehyde is high cytotoxicity and calcification in the host tissue due to incomplete removal of toxic residues [59–61].
Use of various synthetic and biological chemical cross-linkers could address the issue of biocompatibility. Carbodiimides [57, 62, 63], genipin [56, 57], proanthocyanidin [56, 64, 65] and tannic acid [66] are few of the chemicals used for cross-linking collagen. Water-soluble carbodiimide such as l-ethyl-3-(3-dimethyl aminopropyl) carbodiimide hydrochloride (EDC) is commonly used to stabilize and strengthen collagen tissues and scaffolds [57, 63, 67, 68]. Cross-linking using EDC involves the activation of the carboxylic acid groups of glutamic or aspartic acid residues by EDC. EDC and N-hydroxysuccinimide (NHS) can link carboxylic acid and amino groups located within a very short distance of 1.0 nm from each other [69]. This poses a challenge when the functional groups located on adjacent collagen microfibrils are too far apart to be bridged by carbodiimides [67]. The increase in the use of such chemicals, however, results in a decrease in elasticity and toughness as well as biocompatibility to cells.
Proanthocyanidin, a grape seed extract (GSE), cross-linked collagen by covalent interaction, ionic interaction, hydrogen bonding interaction or hydrophobic interaction [70]. Due to these multiple interactions, GSE demonstrates better mechanical properties of cross-linked collagen as compared to the glutaraldehyde. Chemical cross-linking is a time-consuming process, which markedly limits its application in a clinical situation. Cross-linking agents, which are biocompatible or with low cytotoxicity that forms stable cross-linked collagen in a clinically feasible time period, are desired for dental applications.
7.4.2 Physical Cross-Linking
Physical cross-linking is achieved by use of heat, dehydrothermal treatment (DHT) and UV- and gamma- irradiation. The disadvantage of these methods is that they weaken the collagen due to thermal degradation [71] and collagen denaturation [72, 73]. These physical methods also consume hours to days for the cross-linking to take place. So far, these physical methods have not shown significant potential for dental applications [71].
7.4.3 Photodynamic Cross-Linking
Photodynamic therapy (PDT) involves the use of non-toxic dye or photosensitizer in combination with visible light, which in the presence of molecular oxygen leads to the production of cytotoxic oxygen radicals such as singlet oxygen. During PDT, the photosensitizers are capable of transferring the energy absorbed to other compounds, which in turn generate metastable species that are very reactive.
The light excited photosensitizer molecule can release its energy via (1) production of radical ions of oxygen due to electron transfer from photosensitizer triplet excited state to the substrate or (2) production of excited singlet oxygen due to energy transfer from photosensitizer triplet excited state to the ground-state molecular oxygen, which is responsible for the oxidation of various cellular constituents [74]. The latter one is a Type II pathway and is responsible for a majority of the PDT effects including photochemical cross-linking. The highly active singlet oxygen induces photo-oxidation of the amino acids such as cysteine, histidine, tyrosine and tryptophan and leads to the formation of covalent cross-links in a light-independent manner [75]. PDT results in photochemical cross-linking, which strengthened the mechanical properties of collagenous tissues and artificial scaffolds used in tissue engineering [62, 76–79].
Photo-cross-linking of collagen is a rapid process unlike chemical and physical cross-linking processes. In photo-cross-linking, the proteins and collagen molecules are covalently cross-linked (inter- and intramolecular) when illuminated in the presence of appropriate photosensitizers such as Rose Bengal (RB) [53, 80, 81] and riboflavin [77, 78, 82–84]. This close cross-linking results in increased ultimate tensile strength and elastic modulus of collagen. However, there are possibilities for the cross-linked collagen to be very stiff or brittle in nature. Incorporation of synthetic or natural polymers could serve as spacers/fillers in between the collagen fibrils and prevent undesired zero-length cross-linking [76, 85]. This cross-linking combined with the infiltration of polymeric fillers would reinforce the collagen structure, ensuing in the increased post-yield strain and fracture toughness [76, 86–88].
7.5 Nanoparticles and Their Role in Dentin Stabilization
Nanotechnology has been applied to dentistry as an innovative concept for the development of materials and/or treatment strategies. The scope of nanomaterials to eliminate biofilms, inhibit a demineralization process and promote remineralization of tooth structure to combat disease-causing bacteria and repair/stabilize previously diseased dentin matrix is being investigated (Fig. 7.5).
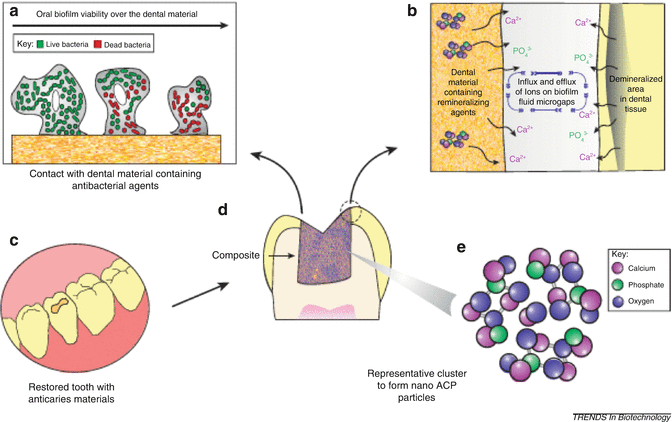
Fig. 7.5
Schematic illustration of the proposed anticaries procedures via restorative dental materials: antibacterial and remineralizing (ion-diffusion process) approaches. (a) The antibacterial approach involves release of nanostructured agents as described in the text. The strong antibacterial action is mainly attributed to the high surface:volume ratio that maximizes contact with the environment. These small particles easily penetrate through cell membranes and affect intracellular processes resulting in higher reactivity and antimicrobial activity. (b) In the remineralizing approach dental materials release calcium and phosphate to the dental plaque fluid present in microgaps between the tooth and restoration. The calcium and phosphate may be deposited into the tooth leading to gain of net mineral. (c) Clinical applicability of nanotechnology-based materials for dental caries management for restoring teeth with cavities. (d) Schematic drawing of a longitudinal section of a restored tooth showing the close contact of dental material with dental tissue. (e) Representative cluster to form amorphous calcium phosphate (ACP) nanofillers (NACP) with detail of molecular components (Adapted from Melo et al. [118]. With permission from Elsevier)
Nanoparticles of various materials (polymers, metals), sizes and shapes as well as modifications are available depending on the requirement or treatment needs. Nanoparticles could be tailored to perform specific or multiple functions based on the requirement of the infected tissue management such as root canal disinfection or minimally invasive management of dental caries. Careful selection of nanoparticles based on sound scientific grounds that encompasses the mechanism of action, safety and clinical requirements of dentin tissue stabilization will benefit endodontics and restorative dentistry.
Nanoparticles of bioactive polymers such as chitosan showed ability to enhance the mechanical properties of dentin collagen. Application of chitosan nanoparticles offers several attractive advantages such as the folowing: (1) They possess structural similarity to the extracellular matrix glycosaminoglycans [89]. Extracellular matrix proteins such as proteoglycans and glycosaminoglycans provide mechanical stability and compressive strength to the collagen by intertwining with the fibrous structure. (2) Chitosan composites with collagen could reinforce the collagen scaffolds as well as create a more suitable biomimetic environment for cells [90]. (3) Chitosan nanoparticles consist of reactive free amino and hydroxyl groups that can be utilized for chemical modifications and conjugation. Other reactive molecules/proteins could be attached to the chitosan nanoparticles to obtain a multifunctional nanoparticle. (4) Chitosan is known to be a non-toxic, biologically compatible polymer allowing its widespread use in biomedical applications. (5) The low solubility at a physiological pH of 7.4 can be taken care of by modification of its functional amino group. Conjugation of chitosan with Rose Bengal resulted in water-soluble particles even at higher pH [91]. (6) Chitosan nanoparticles and their derivatives interact with and neutralize MMPs or bacterial collagenase, thereby improving dentinal resistance to degradation [44, 92].
Nanoparticles of carbonated apatite, silica and bioactive glass have been used for the management of dentinal hypersensitivity [93–95]. Occlusion of exposed and patent dentinal tubules is an effective means to provide relief from dentinal hypersensitivity. The main advantage of using nanoparticles is their size effect, which allows these nanoparticles to be pushed into deeper aspects of the dentin tissue. Furthermore, these nanoparticles once modified or functionalized could promote remineralization of the demineralized dentin or occluded patent dentinal tubules [93, 96]. The topic of nanoparticles for dentinal sensitivity management has been covered in detail in the chapter 8 of this book.
Nanomaterials have been applied to improve the overall efficacy of PDT [97]. Towards this end, the key area of interest is using nanoparticles either in conjugation or in combination with photosensitizers depending on the treatment requirement. The nanoparticle-conjugated photosensitizers such as Rose Bengal–conjugated chitosan nanoparticles (CSRBnps) could be used to stabilize dentin collagen [76]. Rose Bengal–conjugated chitosan in micro- and nanoparticles (CSRBnps) produce singlet oxygen upon photoactivation with a green light (540 nm wavelength). This singlet oxygen produces additional cross-linking between collagen molecules as well as collagen and chitosan nanoparticles. Chitosan incorporation into the photo-cross-linked dentin collagen improved the mechanical properties and resistance to collagenase degradation. In high-magnification images using transmission electron microscopy, CSnp incorporation into the collagen architecture could be evaluated (Fig. 7.6) [76]. CSnp that was incorporated on the dentin collagen could also act as an antibacterial coating, which prevents bacterial ingress along the tooth-filling interface and early biofilm formation. Similar concepts of coating surfaces with chitosan have been tested to reduce bacterial adherence and biofilm formation [98].
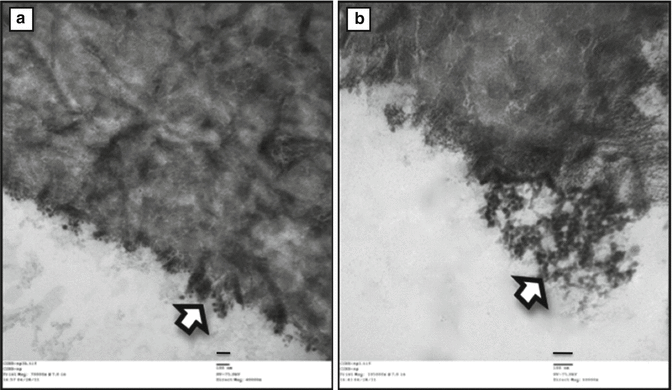
Fig. 7.6
(a, b) Transmission electron microscopy image of dentin collagen following photodynamic crosslinking using photosensitizer conjugated chitosan nanoparticles. Arrow indicate the nanoparticles lining the surface of the dentin collagen (Adapted from Shrestha [76]. With permission from Elsevier)
7.6 Effects of Stabilization on Dentin
Dentin stabilization is known to affect both mechanical properties and chemical characteristics of dentin matrix. Based on the various methods used to achieve dentin stabilization, the final properties of the matrix could vary. The following paragraphs will highlight the effects on specific properties of dentin after cross-linking procedures.
7.6.1 Effects on Mechanical Properties
As mentioned above, each constituent of dentin composite plays a distinct role in its mechanical properties. Collagen fibrils are the principal tensile stress–bearing component of dentin. The resistance to thermal and proteolytic cleavage and structural stability is provided by the high degree of intermolecular cross-linking and a tight mechanical weave [99, 100]. The procedures that involve cross-linking of collagen should typically show effects on the tensile properties and toughness of dentin. However, when the bulk of dentin is considered, even the compressive strength has been found to improve following cross-linking procedures. Dentin collagen cross-linked either with carbodiimides or photodynamic cross-linking using Rose Bengal showed significantly improved tensile strength and toughness. Generally, cross-linking of collagen using different agents increases the tensile properties, elastic modulus, hardness and toughness of demineralized dentin [55, 56, 62, 66, 76–79].
The incorporation of water-soluble chitosan during cross-linking further enhanced the mechanical properties of dentin [62]. The use of multifunctional chitosan-conjugated Rose Bengal nanoparticles helped achieve this photodynamic cross-linking in a single step [76]. CSRBnp-treated dentin collagen revealed marked improvements in the mechanical properties. Similarly, riboflavin is another potent photosensitizer that could produce collagen cross-linking [79, 82, 83] and showed improved mechanical properties of dentin. Proanthocyanidin (6.5 %) extracted from grape seeds showed increase of the elastic modulus from 8 to 40 times after 10 min and 4 h of treatment respectively [55]. The proanthocyanidin-treated dentin resisted the progression of root caries lesion depth to 80 μm as compared to 208 μm depth in untreated cases [101].
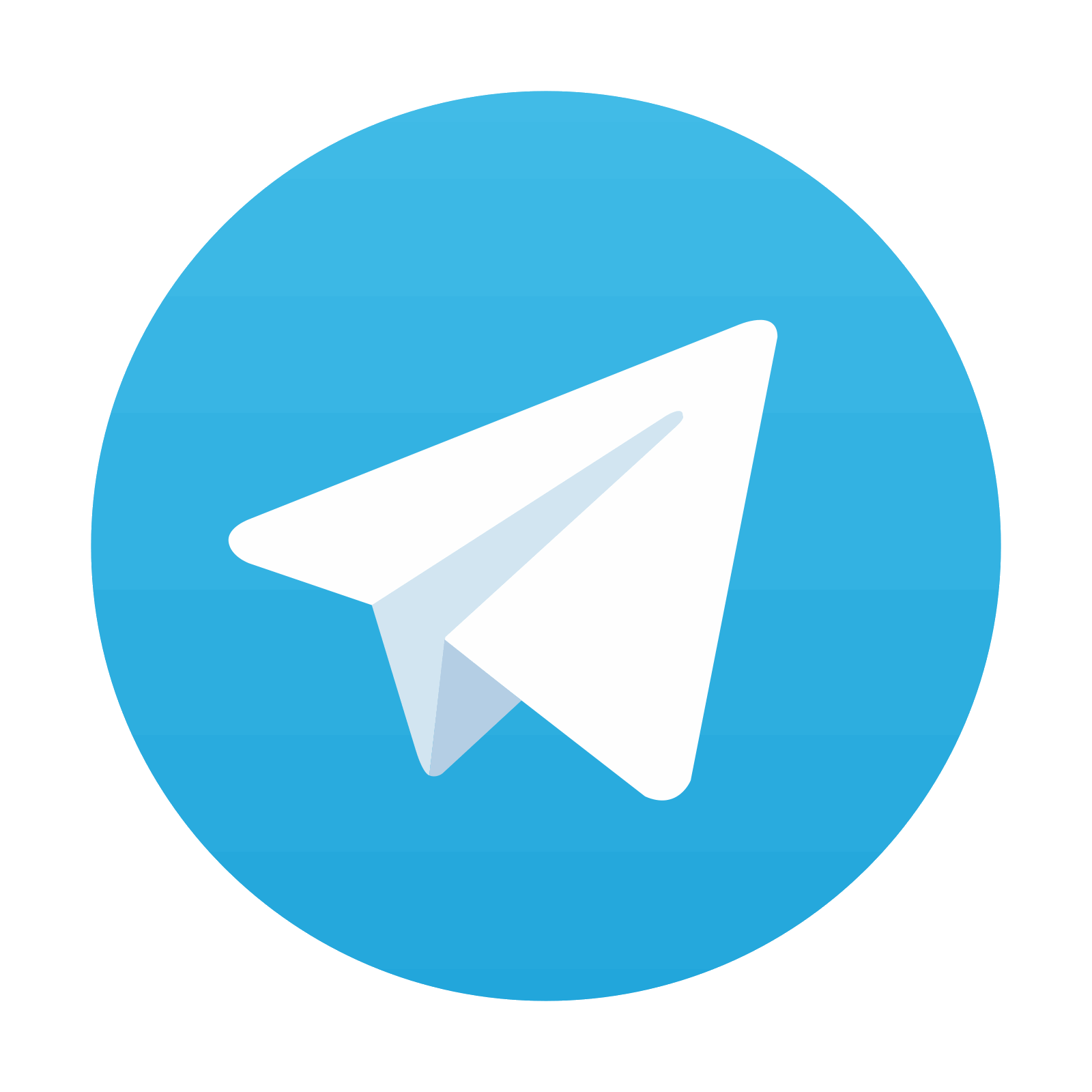
Stay updated, free dental videos. Join our Telegram channel

VIDEdental - Online dental courses
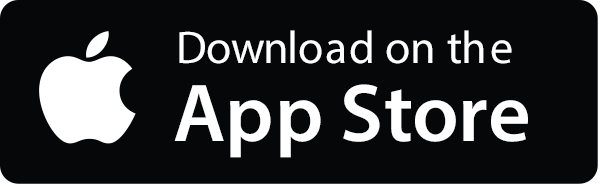
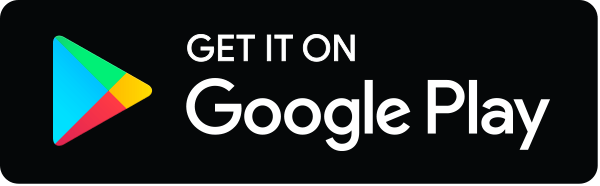