(1.1)
Portland cement differs from these ancient cements because it contains the pre-reacted hydraulic calcium silicate powders rather than hydration of CaO or Ca(OH)2 with silica. Higher compressive strengths were achieved with Portland cements, which supplanted the use of pozzolanic cement by the 1900s. However, pozzolanic materials (fine silica-containing powders) are now added to Portland cement to reduce the cost of Portland cement, improve workability, retard the setting time or reduce the amount of water needed.
For Portland cement, the raw materials, the formula, the phase proportions and the firing and grinding methods vary depending on the local raw materials and are different in every cement factory around the world. The raw materials usually are calcium carbonate blended with silica- and alumina-containing minerals, such as (1) limestone, shells or chalk for calcium oxide; (2) shale, clay, sand, slag for silicon oxide and aluminium oxide; and (3) iron ore. Although the materials vary widely, local raw materials are preferred, particularly the limestone, to keep the costs as low as possible. Although the ternary (three) oxides are sufficient to make a Portland cement, usually 5 % or less of iron oxide or iron is present in the raw materials for reasons explained below. The iron forms a dark-coloured phase, which imparts the usual grey colour to cement. For white Portland cement, the iron content of the raw materials is less than 0.5 %. Chromium, manganese, titanium copper or vanadium or magnesium oxides are also capable of colouring Portland cement. Firing of white cement requires higher temperatures or the additions of fluxes other than iron oxide, such as alumina or sodium and potassium oxides.
The formula for Portland cement is not unique, but covers a range of silica, alumina and calcia compositions. Materials scientists use phase diagrams to illustrate the equilibrium phase relationships of materials, usually over a range of temperatures. Figure 1.1a is a ternary phase diagram for the primary components: silica, alumina and calcia showing the various compounds that can be formed by firing the three oxides in various proportions. The range of Portland cement compositions is the area denoted by “P” in Fig. 1.1b, which includes primarily di- and tricalcium silicate phases and less tricalcium aluminate. Note that monocalcium silicate (CaSiO3, also known as wollastonite) is not a hydraulic (water reactive) phase and is not part of Portland cement in Fig. 1.1.
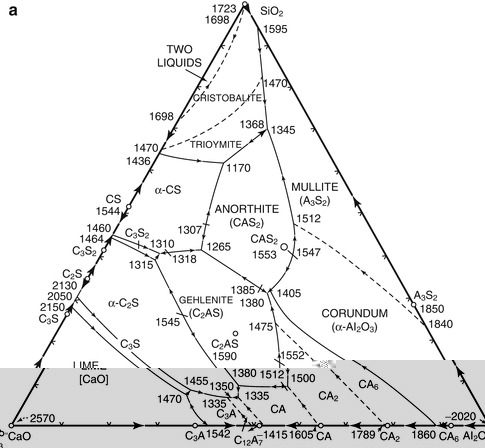
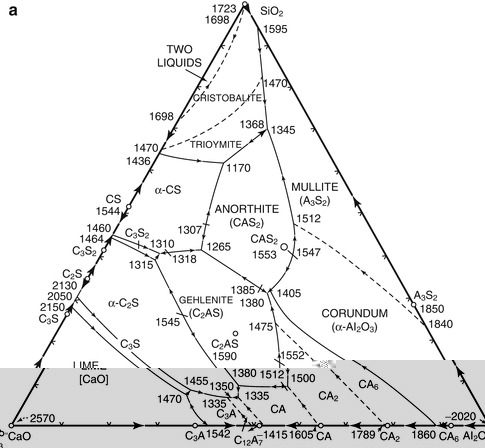
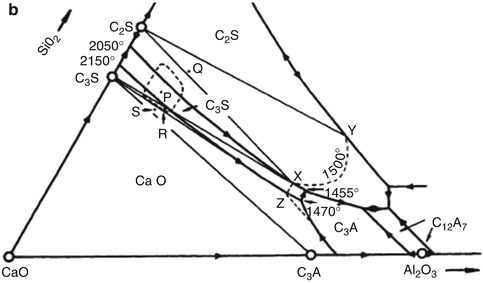
Fig. 1.1
(a) The ternary phase diagram for calcia, silica and alumina showing the many phases that can be formed by reaction of these three oxides at various temperatures (°F). Cement notation (abbreviations) is used for the phases. (b) Section of the ternary phase diagram in (a) showing the range of compositions P where Portland cement is formed of tricalcium silicate (C3S), dicalcium silicate (C2S) and tricalcium aluminate (C3A)
Many standards have been developed for the construction cement industry, from compositional requirements to testing methods and requirements. For instance, Standard Specifications for Portland Cement (ASTM C150) [5] or Cement: Composition, Specifications and Conformity Criteria for Common Cements (EN 197-1) [47] standards for cement compositions restrict the magnesium oxide to less than 5 %. Magnesium oxide is commonly found with calcium compound deposits, just as it is present in the human bone (<5 %) but can expand when hydrated. Barium oxide and phosphorous pentoxide are common trace oxides found with calcium carbonate mineral deposits. Other common accessory oxides in Portland cement are sodium oxide, potassium oxide, titanium oxide, manganese oxide, nickel oxide, phosphorous pentoxide, barium oxide, chromium oxide and fluoride, usually in amounts less than 0.5 % and many at less than 100 ppm. The sodium oxide, potassium oxide, sulphates (from the raw materials or the fuel) and fluoride act as fluxing agents, reducing the firing temperature for Portland cement, which also reduces the cost for making the cement.
The powdered raw materials for Portland cement are blended and usually formed into balls to feed into the kiln. Portland cement is economically manufactured in large furnaces called rotary kilns. During the first part of the firing process (up to about 850 °C), the calcium carbonate decomposes to calcium oxide releasing carbon dioxide, a process called calcining. The calcium oxide is retained in the powder mixture in the kiln and reacts with the silicate, alumina and iron oxide raw materials as the temperature is gradually raised to about 1,500 °C and new phases are formed: calcium silicates and aluminates. Figure 1.2 depicts a typical progression during firing from raw materials to formation of the cement phases. Some intermediate compounds are formed during firing, which react further as the temperature is increased. During firing, as much as 25 % liquid is formed in the material, which solidifies during cooling. This process, called liquid phase sintering, hastens reactions to form the calcium silicate phases and allows the firing temperature to be lower. The firing diagram depicts a starting material that included clay minerals and iron in the raw materials.
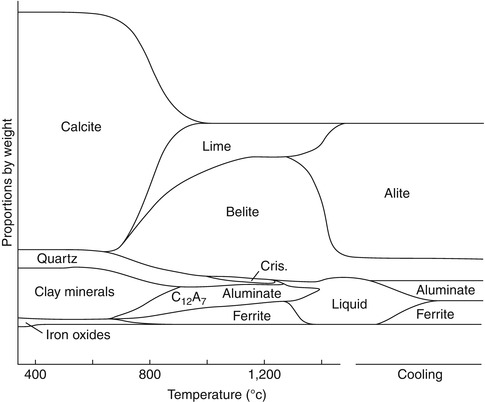
Fig. 1.2
In this diagram depicting firing of Portland cement, the formation of phases is depicted from the raw materials reacting and forming the calcium silicate, aluminate and ferrite phases
The reacted ceramic materials that exit the rotary kilns are large particles (>0.5 cm) called clinker, because of the sound they make when falling into a quenching bin after firing. The clinker nodules are porous and contain several ceramic phases as described below. Rapid cooling is preferred to prevent decomposition of alite to belite and lime (CaO) and to make the grinding easier. Air quenching is usually used to cool the clinker and ensure that only the beta phase of dicalcium silicate (ß-C2S) is formed thus preventing the formation of the gamma phase of dicalcium silicate (γ − C2S). The beta phase is more hydraulic; that is, this crystalline form of the dicalcium silicate more readily forms hydrated C2S, a benefit to the strength of Portland cement.
Keeping the energy and raw materials’ costs low is the primary objective for Portland cement manufacturers, because it is a very price-sensitive commodity. Local materials, lower firing temperature, and minimal grinding are preferred which keep energy and transportation costs lower. Because of the price sensitivity, materials of lesser purity and lower quality fuels are used and grinding is minimized for manufacturing. For instance, worn out automobile tyres are added as a supplemental fuel in firing cement [30]. The raw materials and the fuels can contribute minor amounts of accessory oxides to the composition.
After firing, the clinker particles are crushed and ground to a powder so that most of the cement particles are smaller than 80 microns. Calcium sulphate as gypsum (CaSO4 · 2H2O) is blended with the powder, usually by grinding the materials together (intergrinding). After grinding, the calcium sulphate may be present as the soluble anhydrite (CaSO4) or hemihydrate CaSO4 · ½H2O. Sometimes, other organic grinding aids or CaO are interground. The combined calcium silicate and calcium sulphate powder is called normal or “ordinary Portland cement” (OPC). The degree of grinding determines what type of cement is created; the types are defined in various cement standards including ASTM C150 [5]. Types I and III are the most common with Type III being a finer powder. The calcium sulphate is important for construction because it delays setting reactions in concrete as described below, which is very important for transporting mixed cement and for large concrete structure pouring.
1.3 Portland Cement Phases and Reactions
After manufacture, OPC contains several phases in the powder: alite (tricalcium silicate, C3S), belite (dicalcium silicate, C2S) and a lesser amount of tricalcium aluminate (C3A) and calcium aluminoferrite (ferrite, C4AF) phases. Free lime (CaO) may be present, but preferably in a minor amount, because lime is less hydraulically active. Cement scientists calculate the possible proportions of the cement phases from the raw materials using the Bogue calculation [86].
The alite crystals are very reactive with water and are usually present from 45 to 70 % of the OPC. Belite crystals are less reactive and the reaction is less exothermic; they usually constitute 5–30 % of an OPC powder. Tricalcium aluminate’s hydration reaction is more exothermic than that of alite or belite. Ferrite also reacts with water, but weakly. The tricalcium aluminate and ferrite phases each typically constitute less than 10 % of an OPC. When observed microscopically, the alite crystals are elongated and hexagonal. Belite crystals are more rounded or equiaxed. Ferrite and aluminate phases are usually smaller and attached to alite or belite crystals. Free lime (CaO) forms thin hexagonal plates.
OPC reacts with water to form a solid mass of hydrated gel and unreacted cement particles via a complex and prolonged processes of exothermic setting and hardening reactions. The water to cement ratio is usually 0.3–0.7 by weight. Higher proportions of water generally increase porosity and permeability while decreasing the compressive strength. The reaction products of hydrated phases have been referred to as gels but are now referred to as amorphous reaction products. These processes are governed by the cement’s phase composition, impurities in the phases, fineness of the powder and additions to the cement or water [86]. The cement literature describes the four stages of setting and hydration:
1.
Preinduction, lasting a few minutes
2.
Induction or dormant period, lasting a few hours
3.
Acceleration, about 3–12 h after mixing
4.
Post-acceleration from 12 h onwards
In Stage 1, the calcium sulphate, calcium aluminate and calcium aluminoferrite phases rapidly dissolve, and superficial hydration of the alite phase particles occurs. The calcium sulphate and calcium aluminate form ettringite, a hexacalcium aluminate trisulphate hydrate, of the general formula (CaO)6(Al2O3)(SO3)3·32H2O, also written as (CaO)3(Al2O3)(CaSO4)3·32H2O. Iron can substitute partially for the alumina, and carbonate can partially substitute for sulphate. These needle-like crystals grow in the liquid between particles. Without calcium sulphate, the tricalcium alumina hydrates rapidly, releasing heat, and the hydration causes the cement to become unworkable by quickly reacting with the water. This phenomenon is denoted as “flash setting”. A similar phenomenon of false setting occurs when the sulphate is present as gypsum, not the anhydrite or hemihydrate. When hydration starts, the gypsum may start to precipitate quickly which gives the appearance of setting.
As the cement enters the acceleration stage, both the alite and belite react, as does the formation of ettringite. During Stage 1 or 2, the cement can be “remixed”, which breaks up the nascent hydration structure. During Stage 2 the cement “sets”, which is a gradual transition in cement from a fluid to a rigid state. Setting time is arbitrarily defined by laboratory testing procedures. Initial and final setting tests are described for cement, which are usually determined by using weighted needles to penetrate the cement surface, with either Vicat or Gillmore apparatus, as described later. This stiffening of the cement is a result of the water becoming part of the reaction products on the cement particles that begin to impinge on one another on the microscopic level.
Hydration of the alite crystals proceeds in Phase 3 reducing the free water, and more calcium hydroxide precipitates from the liquid. The hydration reaction for the tricalcium silicate phase that began in Phase 1 resumes following Eq. 1.2:

(1.2)
As the amount of non-hydrated material declines, hydration becomes a slower, diffusion-controlled process of the alite and belite particles. Some of the ettringite crystals dissolve to release tricalcium aluminate and calcium monosulphate (3CaO·Al2O3 + CaSO4·12H2O).
During Stage 4, the belite phase continues hydration following Eq. 1.3, forming the same surface reaction product as alite but releasing less portlandite:

(1.3)
Slow hydration continues at a decreasing rate, and the terminal amount of hydration is usually reached after about 4 weeks. Unreacted cement particles may remain in the solidified mass, each surrounded by a layer of hydrated reaction products. These hydration reactions occur minimally in the presence of moisture in the air. Hence, storing cement in bulk and protected from moisture is advantageous.
1.4 MTA and Portland Cement
MTA was invented by combining a grey Portland cement with bismuth oxide and used for endodontic applications [87, 90]. The original MTA materials contained the same tri- and dicalcium silicate major phases as Portland cement, with about 20 % bismuth oxide. Also, the original MTA products were expected to perform very similarly to OPC in setting, strengthening and hydrating, as was shown by Islam [58]. Unfortunately, the original description in the dental literature was erroneous and contained a description of MTA after reaction with water as [88]:
calcium oxide and calcium phosphate …. The principle compounds present in this material are tricalcium silicate, tricalcium aluminate, tricalcium oxide, and silicate oxide. In addition, there are small amounts of a few other mineral oxides that are responsible for the chemical and physical properties of this aggregate. Bismuth oxide powder has been added to make the aggregate radiopaque. Electron probe microanalysis of MTA powder showed that calcium and phosphorous are the main ions present in this material.
This often quoted description is wrong from a chemical point of view because no compound exists known as tricalcium oxide, and silicate oxide is customarily written as silica or silicon oxide. As a result, this first article on the physical properties of MTA has confused other researchers who have examined MTA products [8]. Errors in the literature about the MTA material’s compositions can be attributed to the researchers using only energy-dispersive spectroscopy (EDS) without confirmatory X-ray diffraction. EDS and energy-dispersive X-ray analysis (EDXA) (electron microprobe analysis) are techniques to identify the characteristic X-rays from the elements present in a material, but these techniques do not identify the compounds (phases). Frequently, researchers have reported the weight percentages of silicon oxide, calcium oxide, aluminium oxide and other metal oxides from EDS [61], EDXA [8], XRF or ICP tests but ignored the apportionment of the oxides into the crystalline phases such as tri- and dicalcium silicate, tricalcium aluminate or the presence of calcium carbonate. Although it is convenient to detect elements with an SEM equipped with EDS, the distribution of the phases by X-ray diffraction is equally important. Knowledge of the crystalline phases is essential because the phases determine the properties of the material and the body’s response. Without knowing the compounds, a discussion of energy-dispersive X-ray spectroscopy (EDS) data is as erroneous as describing salt as sodium oxide and chlorine rather than sodium chloride. The X-ray diffraction analyses of MTA and the appropriate crystalline phases present have been reported [10, 19, 24, 57].
The initial reports from the MTA introduction period into the dental market stated that the material had a basic calcium and phosphorus composition [88]; currently, it is scientifically well established that MTA is comprised of about 80 % Portland cement, which has only trace amounts of phosphorous. The material’s biocompatibility was regarded as a direct consequence of its chemical similarity with hard dental tissues [88]. However, later, it was correctly published that MTA was primarily comprised of tricalcium and dicalcium silicates [24], based on its composition including 80 % Portland cement.
In 1999, the Journal of Endodontics published an abstract of the study presented at the annual meeting of the American Association of Endodontists (AAE), in which MTA was experimentally compared to Portland cement [93]. In this study, MTA’s and Portland’s cement chemical composition and biocompatibility were analysed, and comparable results were found. This was the first time that MTA was scientifically compared to Portland cement. In the following year, Estrela et al. [45] published the first full study in which MTA was directly compared to Portland cement, concluding that both materials were chemically similar, apart from bismuth oxide (the radiopacifier agent) present in MTA. Moreover, Portland cement and MTA were reported to have similar pH and antibacterial capacity. In the following year, Holland et al. [51] also observed similar results between MTA and Portland cement on direct pulp protection of dog’s teeth.
After initial reports, various studies were published comparing MTA with Portland cement. The main reasons behind these research efforts were the high price ($50 per gram) of the only MTA product, ProRoot® MTA from Dentsply Tulsa Dental, USA, and the possibility of developing a low-cost alternative repair cement. Spångberg [83] summarized the situation well in 2006, when he stated that “ProRoot MTA (Dentsply, Johnson City, TN) is a new material, but for practical purposes is not very different from Portland cement. The factor responsible for the beneficial effects in ProRoot is also found in Portland cement”. At the same time, concerns arose about the possible toxic metal content of Portland cement and MTA. However, Spångberg [83] wrote that “Considering the number of uncontrolled toxic materials dentists are allowed to use clinically, such as formaldehyde, cresol, mercury, phenol, eugenol … to name a few, less than half a gram of Portland cement seems like an innocuous amount”. Complaints about the poor handling, slow setting and high price persisted, and many articles were written comparing Portland cement with ProRoot MTA or MTA-Angelus products (Angelus, Londrina, Brazil). Chapter 7 describes the properties of these materials in more detail. Some tested the clinical use of Portland cement [38] as an apical plug in the treatment of an open apex tooth with apical radiolucency. The clinical and radiographic follow-up showed treatment success (Fig. 1.3a–d).
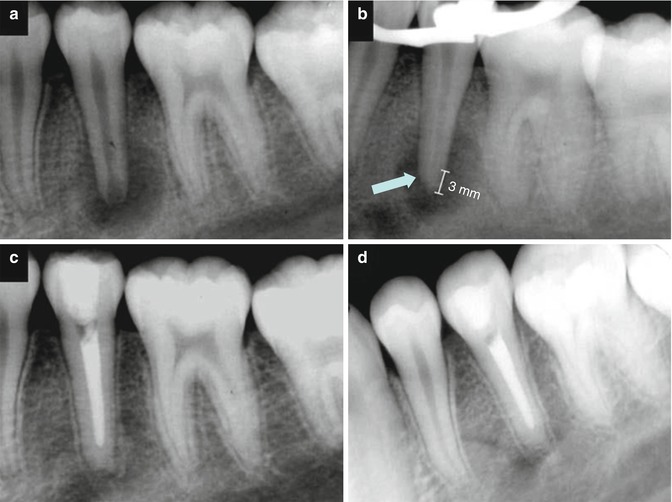
Fig. 1.3
Case report showing the use of white Portland cement as an apical plug in a tooth with a necrotic pulp and wide-open apex. (a) Preoperative radiograph of the mandibular left premolar. Note the wide-open apex and periapical radiolucent lesion. (b) Preoperative radiograph with WCP placed at the apical portion of the canal (approximately 3 mm). (c) Immediate postoperative radiograph with root canal filling and white Portland cement in the apical third. (d) One-year postoperative radiograph confirming healing of the periapical region
More than 150 studies compared MTA to Portland cement, reinforcing the similarities with the exception of bismuth oxide present in the first two MTA products. Thus, it can be concluded that MTA is a kind of Portland cement especially produced for dental use [8, 24, 45, 58]. Additionally, it was ascertained that MTA has less iron-3 (Fe3) and aluminium compounds than Portland cement [34]. Another difference between the two materials is the particle size; MTA has smaller and more regular particles than common Portland cement [34, 59].
Several researchers have raised concerns about heavy metal contamination of both grey and white MTA, believing that the arsenic or lead levels exceeded those permitted in ISO 9917-1:2007 for powder/liquid acid–base dental cements [55], namely, 2 or 100 ppm of acid-extractable arsenic or lead. These concerns arise from comparisons to construction-grade Portland cements, which are known to contain metal oxides including arsenic. Various construction-grade Portland cements have been tested as a substitute for MTA products because MTA is expensive when compared to other dental cements [1, 7, 58]. MTA is claimed to be produced under controlled conditions, resulting in a pure and well-controlled hydraulic cement powder, composed of contamination-free biocompatible particles. This is an important issue, since repair cements are classified as permanent-contact implant devices with the potential of causing damage or irritation of the periapical tissue and delaying wound healing.
Studies have measured the total (not acid-extracted) arsenic (Table 1.1) in Portland cements and MTA products and found amounts that exceed the ISO 9917-1:2007 [55] limits. However, white Portland cement and white MTA have lower arsenic contents than their grey counterparts. Overall results of the heavy metals in both MTA and some Portland cement brands are negligible [81] and less than 10 ppm. Moreover, it must be understood that although hydraulic cements may have higher amounts of contaminants than those established by ISO 9917-1:2007 [55], leaching in solution is low (Table 1.2) [42, 81] as arsenic oxide is dissolved in the silicate and is relatively insoluble [39].
Table 1.1
Amount of arsenic in MTA and some commercial brands of Portland cement
Material
|
Arsenic (mg/kg) or (mg/g)
|
---|---|
CPM
|
11.06
|
CPM sealer
|
10.30
|
MTA-Obtura
|
0.39
|
MTA-Experimental
|
10.13
|
White MTA-Angelus
|
1.03
|
Grey MTA-Angelus
|
5.91
|
ProRoot MTA
|
5.25
|
Grey Portland cement
|
34.27
|
White Portland cement
|
0.52
|
Table 1.2
Arsenic release (ppm) by MTA and some commercial brands of Portland cement
Votoran
|
Ribeirão
|
Irajá branco
|
ProRoot
|
MTA Angelus
|
||||||
---|---|---|---|---|---|---|---|---|---|---|
P
|
3 h
|
168 h
|
3 h
|
168 h
|
3 h
|
168 h
|
3 h
|
168 h
|
3 h
|
168 h
|
M
|
0.0007
|
0.0003
|
0.0002
|
0.0002
|
0.0002
|
0.0002
|
0.0002
|
0.0002
|
0.0002
|
0.0002
|
DP
|
0.0006
|
0.0002
|
0
|
0
|
0
|
0
|
0
|
0
|
0
|
0
|
Portland cement powder has insufficient radiopacity (<3 mm equivalent Al) [54] for dental materials as required in ADA 57 [4] and ISO 6876 [56] standard specifications. MTA’s radiopacity was first achieved by blending bismuth oxide with the tricalcium silicate powder. ProRoot MTA has on average 7.5 mm Al [20], while MTA-Angelus has about 5.7 mm Al [17]. Lower radiopacity is attributed to less bismuth oxide in MTA-Angelus with the latter having 14 % bismuth oxide [27] as opposed to ProRoot MTA, which contains 20 % [10, 19]. Larger particle sizes or poorer dispersion also causes variations in radiopacity. The radiopacifier may affect the hydration and final properties of the cement. Bismuth oxide reduced compressive strength and increased porosity, as well as diminished the cellular growth [26, 32]. Leaching of bismuth in solution has also been reported [20]. Furthermore tooth discolouration has been associated with bismuth oxide in MTA [11]. Studies have tested the radiopacity imparted to raw Portland cement by higher molecular weight additions of gold and silver [20], zirconium oxide, calcium tungstate, zinc oxide, iodoform and barium sulphate [20, 54] (Table 1.3). Zirconium oxide has been studied as an alternative radiopacifing agent with the advantage of not affecting cement hydration as well as improving cement mixture homogeneity and consistency [22]. However, its atomic number is rather low compared to the usual dental radiopaque agents.
Table 1.3
Radiopacity (mm Al) of dentin and pure Portland cement and its association with different radiopacifing agents
Material
|
Average
|
SD
|
---|---|---|
Portland cement + bismuth carbonate
|
3.25
|
± 0.38
|
Portland cement + iodoform
|
4.24
|
± 0.32
|
Portland cement + bismuth oxide
|
5.93
|
± 0.34
|
Portland cement + lead oxide
|
5.74
|
± 0.66
|
Portland cement + zinc oxide
|
2.64
|
± 0.02
|
Portland cement + zirconium oxide
|
3.41
|
± 0.19
|
Portland cement + barium sulphate
|
2.80
|
± 0.18
|
Portland cement + bismuth subnitrate
|
4.66
|
± 0.42
|
Portland cement + calcium tungstate
|
3.11
|
± 0.25
|
Pure Portland cement
|
1.01
|
± 0.01
|
Dentin
|
1.74
|
± 0.02
|
Similar results have been measured for the pH and calcium release by MTA and Portland cement [20, 58]. Portland cement demonstrates a higher calcium release ability than MTA due to the higher percentage of tricalcium silicate [18, 19, 73]. Moreover, it is important to note that biomineralization phenomenon (formation of hydroxyapatite in vivo) of both MTA and Portland cement has been demonstrated [76] (Fig. 1.4
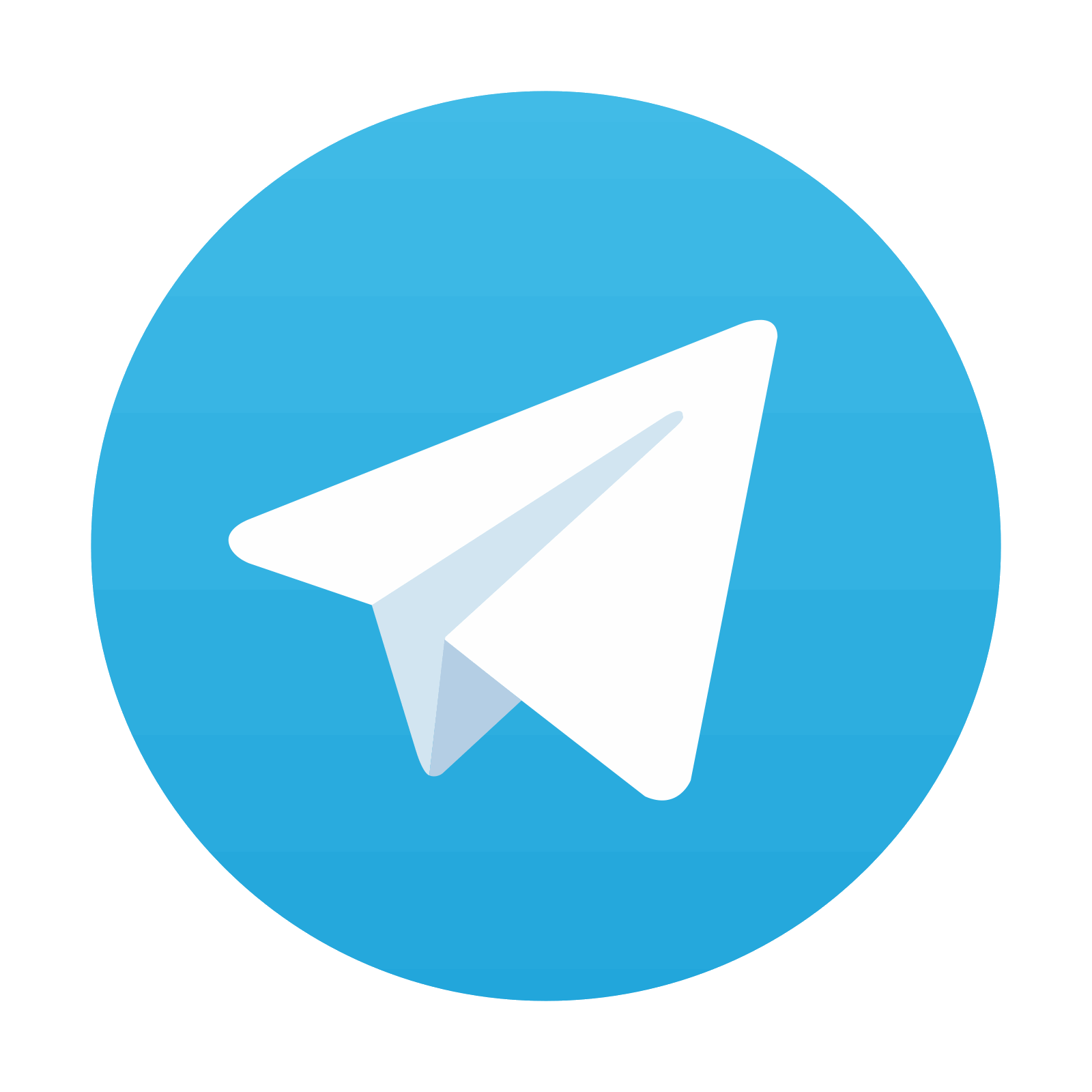
Stay updated, free dental videos. Join our Telegram channel

VIDEdental - Online dental courses
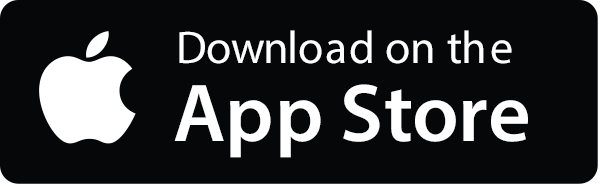
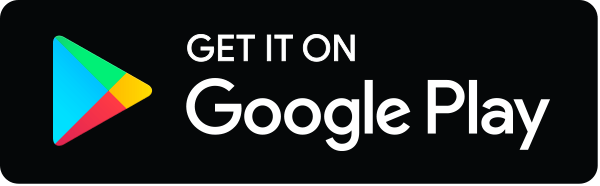