9
Craniofacial Regeneration—Bone
Introduction
Bone tissue engineering is a multidisciplinary field that includes biology, medicine and engineering areas, of which the main objective is bone regeneration. The craniofacial defects are challenges because of their complicated structure and function, the various etiological factors are trauma, tumor or cyst resection, infectious diseases, and also the congenital and developmental condition.
Therefore, it is important to know the anatomical and physiological structures of the craniomaxillofacial bone for further understanding of the complex bone regeneration and repair of normal bone tissue, in order to find new alternatives to improve the treatment used to date in the clinic (autografts, allografts, xenografts and synthetic substitutes), such as 3D printing or scaffolding manufacturing that meet the characteristics of biocompatibility, osteogenicity, osteoconductivity, osteoinduction and osseointegration. The ideal bone substitute should replicate the essential traits of the native bone and evaluate the metabolism and microarchitecture through molecular imaging technology.
Generalities of the Craniofacial Structure
Anatomy
Craniofacial bone tissue (CF) is a complex physiological structure consisting of bone and soft tissue (Gaihre et al. 2017; Datta et al. 2017). The bone tissue provides essential structural support and projection to overlying soft tissue structures such as tendons, ligaments, muscles, facial skin, nerves, blood vessels and sensory organs (Kawecki et al. 2018; Visscher et al. 2017).
The craniofacial bone can be divided according to their functions and structures in the neurocranium, face skeleton and oral apparatus (Fig. 9.1).

Figure 9.1. Craniofacial anatomy, front and lateral view of the cranial.
The neurocranium encloses the brain, the 12 cranial nerves, and the vascular supply within the brain. Its primary function is to protect the brain and is composed by eight bones: the frontal, ethmoid, sphenoid, two parietal, the occipital and the temporal bones (Chu et al. 2014). The face skeleton is defined by 14 bones: two palatine bones, two lacrimal bones, the maxilla bones, the mandible, the vomer, the zygomatic, two inferior nasal turbinates, as well as two nasal bones (Gaihre et al. 2017; Kawecki et al. 2018).
The oral apparatus includes both soft and hard tissues. The soft tissues are the hard tissues comprising the alveolar bone, the alveolar process and the teeth, which include three structures: the enamel, cementum and dentine. The soft-tissue component is the pulp (Gaihre et al. 2017; Chu et al. 2014). All the above-mentioned bones have specific shapes, different volumes and provide a frame on which the soft tissues of the face can act to facilitate facial expression, eating, breathing and speech. These key characteristics will have to be considered when selecting the ideal graft for craniofacial reconstruction (Kawecki et al. 2018).
Neovascularity
Bone is a richly vascularized connective tissue, the process of neovascularization plays a significant role in the process of bone development (endochondral and intramembranous ossification), regeneration and remodeling, which involves both angiogenesis and vasculogenesis (Fishero et al. 2014; Filipowska et al. 2017).
Blood vessels of the bone develop through the process of angiogenesis, which involves the proliferation of local endothelial cells to produce new blood vessels from pre-existing vessels in a remodeling process. The vasculogenesis is the formation of a vascular network, from a progenitor cell, angioblast or hemangioblast. The blood vessels supply the bone system with nutrients and oxygen, excrete waste biological materials, remove metabolites from the bone, provide the bone with specific hormones, growth factors and neurotransmitters secreted by other tissues, maintaining the bone cells survival and stimulating their activity. The craniofacial bones develop by two processes: intramembranous ossification and endochondral ossification. Intramembranous ossification is the main mechanism leading to a development of flats bones (e.g., maxillae, palatal bones, nasal bones, zygomatic bones) this process is related to a direct differentiation of mesenchymal stem cells into osteoblasts, initially with a fibrous membrane and finally replaced by a spongy bone, whereas the endochondral ossification is typical of long bones and the cranial base, this process has an intermediate stage with cartilage (Chu et al. 2014; Fishero et al. 2014; Filipowska et al. 2017). The development and maintenance of the endochondral and intramembranous bone formation are dependent on the bone vascular network (Filipowska et al. 2017; Prisby 2017).
The bone is a dynamic tissue which is in a continuous process of remodeling. Bone remodeling is performed by groups of cells called Bone Multicellular Units (BMU) (Fig. 9.2), the main characters of this process are osteoclasts (bone-resorbing) recruited when needed from their cell precursors, osteoblasts (bone-forming) biosynthesized of/by new bone to replace the former is carried out by osteoblasts and osteocytes that are mechanosensors which monitor mechanical stress within bone tissues (Visscher et al. 2017; Prisby 2017; Arias et al. 2018).

Figure 9.2. BMU: Main molecules induced in the process of remodeled bone. aOCY: apoptotic osteocytes, OCY: Osteocytes, OBA: activated osteoblasts, OBL: osteoblasts, OCL: osteoclasts (modified from Arias et al. 2018).
Remodeling has basically four stages, the activation phase; in which initiation with the osteocyte cell death is caused by bone microdamage, traumatic bone fractures, the decreased sclerostin levels active in BMU, in this phase the osteoclasts precursor cells are recruited to the altered bone surface, alert signals are produced for recruit immune cells and the mediator’s inflammatory process such as Vascular Endothelial Growth Factor (VEGF), angiopoietins, HMGB-1 and cytokines such as IGF-1, growth factors such as Transforming Growth Factor-β (TGF-β) induce osteoblast differentiation, basic Fibroblast Growth Factor (bFGF) and PDGF activate osteoblast and inhibition osteoclast action, Platelet-Derived Growth Factor-BB (PDGF-BB), and Inulin-like Growth Factor-I (IGF-I) contribute to the induction of callus formation, released from bone matrix, and then activating osteoblast differentiation. Next is the resorption phase, when the mature osteoclast degrade the mineralized matrix, the main signals in this step are Macrophage Colony Stimulating Factor M-CSF and ligand Receptor Activator of Nuclear Factor kappa-B RANKL they promote the differentiation of osteoclasts precursors; following the reversal phase, activated osteoblasts advance in the wake of bone-destroying cutting cones to replenish the cavity left behind by the latter, the osteoblasts are recruited and the osteoclasts undergo apoptosis; later during the formation phase, where the osteoblasts lay down new organic bone matrix that subsequently mineralizes, some of the active osteoblasts become trapped in the matrix that they secrete and subsequently differentiate into mature osteocytes. All these phases together contribute to the formation of a complete remodeled bone which is both structurally and functionally similar (Langdahl et al. 2016; Arias et al. 2018).
Etiologies of the Craniofacial Bone Defects
The craniofacial bone defects are challenges for tissues engineering because of the presence of complex physiological structures, including cartilage, facial skeletal features, muscles, skin, ligaments, blood vessels and nerves.
The bones of the craniofacial skeleton provide an essential role in supporting the adjacent soft tissues, providing anchorage for dental structures, maintaining structural stability for many physical functions and composing the aesthetics of the human body.
There are various conditions or etiologies for craniofacial defects such as congenital malformation, cleft palate defects, trauma, infections and tumor or cysts resection, often leading to large psychomedical burdens as well as difficult reconstructive (Wan et al. 2006; Ward et al. 2010; Si et al. 2015; Datta et al. 2017). In this chapter, we will briefly describe the most important etiologies of craniofacial defects.
Congenital Anomalies and Disorders
The most common birth congenital anomalies, include orofacial clefts, craniosynostoses, the mandibulofacial dysostoses and craniofacial macrosomia. Congenital anomalies occur in approximately 3 to 5% of all live births. Furthermore, many of these conditions have a genetic etiology such as chromosomal, single-gene disorders or epigenetic mutation or may be caused by teratogens (Saal 2016).
Whitaker proposed a classification of craniofacial anomalies according to a major structure involving (the lip and palate, eyes, nose, mandibular and maxillary abnormalities) on etiology and treatment principles (Pashayan and Reichman 1980) see Table 9.1.
Most recognizable craniofacial syndromes are monogenic Mendelian disorders, different mutations in the same gene. However, it is not a rule because, more than 7000 single-gene disorders have been identified in craniofacial disorders such as Stickler syndrome and the craniosynostosis syndromes involving different FGFR genes (FGFR1, FGFR2, FGFR3).
In genetic medicine, there are a growing number of clinically indistinguishable or overlapping phenotypes of craniofacial disorders that may be caused by mutations in different genes (locus heterogeneity). These include rasopathies, cohesinopathies, mandibulofacial dysostoses and Stickler syndrome, whereby differential diagnosis is crucial for treatment (Sanchez-Lara 2015).
Table 9.1. Whitaker classification of craniofacial anomalies.
Type |
Definition |
|
I |
Clefts |
Centric (surrounding structures are displaced laterally, require reposition) • Facial clefts • Cranial extensions Acentric • Facial clefts • Cranial clefts |
II |
Synostoses |
Symmetric • Metopic • Coronal • Sagittal Asymmetric • Unilateral coronal closure • Lambdoid closure |
III |
Atrophy (hypoplasia) |
Atrophy of skin, subcutaneous tissue, muscle, bone (e.g., Romberg syndrome, coup the sabre) |
IV |
Neoplasia (hyperplasia) |
Lymphangioma, hemangioma, fibrous Dysplasya |
V |
Unclassified |
Multiorgan involvementSingle-organ involvement |
Modified from Buchanan, P.E., Xue, S.A. and Hollier Jr, H.L. 2014. Craniofacial syndromes. Plastic and Reconstructive Surgery 134: 128–153.
Cleft lip with or without cleft palate (CLP) is among the most common birth defects caused by genetic and nongenetic factors. Several genes could be implicated in CLP; IFR6, TGF-A, TGF-B3 and MSX1 (Howard et al. 2008). Nongenetic factors may include, fetal environment, teratogenic exposures, placental factors and the health of the mother.
Maternal illnesses could be because of congenital craniofacial disorder, the greatest risks associated with type 1 diabetes mellitus are a cleft lip, Cleft Palate (CP), and Pierre Robin Sequence (PRS). On the other hand, illness relating to craniofacial anomalies are phenylketonuria affecting women who do not follow a phenylalanine-restricted diet. The elevated levels of the metabolites of phenylalanine can cause multiple anomalies, including microcephaly, ear anomalies, congenital heart defects and CP.
The craniosynostosis have been associated with maternal hyperthyroidism and serious diseases (Howard et al. 2008).
Other etiologies cause craniofacial congenital anomalies are teratogens which are exogenous substances or physical agents, there are many teratogens such as tobacco, medications (nitrofurantoin and warfarin used during pregnancy), infectious agents, physical agents, radiation, alcohol, toluene and cocaine. The main anomalies relation with teratogens are microcephaly, brain anomalies, holoprosencephaly, limb anomalies, short stature, and behavior disorders, also including CLP, CP, and PRS (Saal 2016; Nagy et al. 2014).
Trauma
One common cause of craniofacial defects is trauma including acute trauma, falls, assaults, sport injuries and vehicle accidents (Zhang and Yelick 2019).
According to Detroit Medical Center (DMC) records 30,260 adult facial fractures were identified, these included nasal (30.1%), mandible (22.7%), malar-maxillary (15.4%), orbital floor (15.7%), and other (16.1%) fractures.
Facial injuries are more common in men than women (68% compared with 32%), furthermore half of all the patients were between 15 and 45-years-old (Walker et al. 2011). Different authors mention other causes of craniofacial defects such as intoxication with alcohol, illegal drugs, Motor Vehicle Accidents (MVAs) in adults. However, children account for approximately 14% of all facial fractures, three trauma mechanisms of pediatric facial fractures: MVA (43%), Intentional Trauma (IT) (17%) and falls (11%) the incidence of inpatient facial fractures due to MVA and IT increases with age. Nevertheless, African American and Hispanic patients accounted for most patients in the IT group and came from the poorest neighborhoods (Streubel and Minsky 2016).
Treatments for Craniofacial Bone Defects
Current surgical treatment for craniofacial defects has been improved during the last year. Nowadays various techniques have been used (autogenous grafting, allogeneic grafting, and prosthetic materials) for bone reconstruction. However, tissues engineering has played a crucial role in bone regeneration for craniofacial defects, as they are common critical size defects and their complicated structure and function. However, despite the use of grafting, none of these modalities have yet to prove a consummate tool for craniofacial bone reconstruction, therefore the emergence of tissue engineering shows great potential as a future treatment for craniofacial defects as tissue engineering must consider three main factors to achieve bone regeneration such as cells, scaffold and growth factors, which influence cellular activity. All of them have proposed one which mimics the extracellular matrix of tissues.
Here the principal treatments for bone regeneration of craniofacial defects, including grafting, scaffolds, cells, growth factor, etc., are described.
Grafting
Bone grafting is one of the most commonly used surgical methods to augment bone regeneration, and the second most frequent tissue transplantation just after blood transfusion. Bone grafts differ in terms of their properties of osteoconduction, osteoinduction, osteogenesis and structural support. As a result, in order to identify the ideal graft, surgeons should understand the requirements of the clinical situation and of the specific properties of the different types of bone graft. Among available grafts, autografts and allografts are considered the best approach However, these strategies are associated with their own disadvantages, including limited availability in case of autografts and potential immunogenic rejection when it comes to the utilization of allografts (Gaihre et al. 2017; Wang and Yeung 2017; Fillingham and Jacobs 2016).
Other forms of bone grafts, such as xenografts and synthetic grafts, eliminate the need for secondary procedures and obviate donor site complications, which make Bone Grafts and Substitutes (BGS) among the most promising in the orthopedic industry. We also describe the most common bone graftings (Shibuya and Jupiter 2015; Wang and Yeung 2017).
Autografts
Autologous bone is still considered as the gold standard since all the necessary properties required in bone regeneration are present, it holds viable cells that can form new bone tissue (osteogenic), it provides a scaffold for the ingrowth of cells necessary for bone regeneration (osteoconductive); and promotes the proliferation of stem cells and their differentiation into osteogenic cells (osteoinductive). It is ideal in many situations because it is harvested from the patient himself or herself, thus less likely to be rejected and more likely to be incorporated. However, the use of an autograft has limitations, including donor site morbidity, limited availability of tissue, an additional operation and prolonged healing time (Henkel et al. 2013). The major and minor complication rates of autogenous bone graft harvest have been reported at 8.6 and 20.6%, respectively (Fillingham and Jacobs 2016).
Other forms of bone grafts, such as allografts, xenografts and synthetic grafts, eliminate the need for secondary procedures and obviate donor site complications. However, rejection and slower incorporation can be disadvantages to the use of these grafts. In well-vascularized bones, such as the calcaneus, it has been documented that there is no difference in the complication rate at the osteotomy site between autograft and allografts. Nevertheless, in less vascularized areas, incorporation can be difficult.
Allografts
Allografted bone is harvested from human donors, mainly cadavers. Its ready availability in various shapes and sizes, avoidance of the need to sacrifice a host structure and no donor-site morbidity are some of the advantages. The disadvantages include infection such as transmission of Human Immunodeficiency Virus (HIV), Hepatitis C Virus (HCV), Human T-Lymphocytic Virus (HTLV), unspecified hepatitis, tuberculosis and other bacteria has been documented for allografts (mainly from those containing viable cells) and immune resistance (Henkel et al. 2013).
Allografts are procured from humans and undergo vigorous sterilization processes before they are ready for surgeons to use. They can be prepared using a combination of different processing procedures. Cadaveric allograft bone is available in either cancellous or cortical forms, or as a Demineralized Bone Matrix (DBM). Allografts are primarily osteoconductive, while DBM is processed in such a way as to retain osteoinductive properties (Fillingham and Jacobs 2016).
In general, allogenic bone grafts can be classified into fresh, fresh-frozen, freeze-dried and demineralized types, depending on the preparation process. Although a more vigorous sterilization process can eliminate the chances of disease transmission and infection, it can also reduce osteogenic and osteoinductive properties. In general, fresher grafts are more expensive and less readily available than other grafts that have a longer shelf life.
Xenografts
A xenograft comes from a nonhuman species. They are materials with their organic components totally removed, so concern about immunological reactions becomes nonexistent. Therefore, antigenicity is significantly greater than that of allografts. The remaining inorganic structure provides a natural architectural matrix as well as an excellent source of calcium. The inorganic material also maintains the physical dimension of the augmentation during the remodeling phases (Hoexter 2002). Naturally, it requires more sterile processing, which can result in reduced osteoinductive properties. However, owing to the abundance of donors, these grafts may be less expensive and more readily available Also because of the extensive sterilization processes, the shelf life is generally long. The most common xenogenous bone graft used in orthopedic surgery is porous natural bone hydroxyapatite from animal bones (bovine, equine, porcine, etc.), are also part of this group. Phytogenic materials such as bone-analog calcium phosphate originally obtained from marine algae or coral derived materials, also fall into this category (Wang and Yeung 2017; Shibuya and Jupiter 2015; Henkel et al. 2013).
Bone Graft Substitute Materials (BSM)
Bone substitutes can be defined as “a synthetic, inorganic or biologically organic combination—biomaterial—which can be inserted for the treatment of a bone defect instead of autogenous or allogenous bone” (Henkel et al. 2013), an ideal bone substitute material should offer an osteoinductive three-dimensional structure, contain osteogenic cells and osteoinductive factors, have sufficient mechanical properties and promote vascularization.
BSM is classified according to their origin (Diagram 9.1).

Diagram 9.1. Bone grafts substitute materials (BSM).
Synthetic Materials (Alloplastic)
Artificial bone can be created from ceramics such as calcium phosphates, bioglass and calcium sulfate that are biologically active depending on solubility in the physiological environment. The varying nature of this graft material (porosity, geometries, differing solubilities and densities) will determine the resorption of calcium phosphate-based graft materials, bioceramics are neither osteogenic nor osteoinductive, but work by creating an osteoconductive scaffold to promote osteosynthesis. Today there are four main types of bioceramics available: calcium sulfate, calcium phosphate, tricalcium phosphate and coralline hydroxyapatite; composite bioceramics use a combination of these types to provide materials with improved properties (Kumar et al. 2013; Martin and Bettencourt 2018; Fillingham and Jacobs 2016).
Titanium metal also belongs to this group. Moreover, polymers including polymethylmethacrylate (PMMA), polylactides/polyglycolide and copolymers as well as polycaprolactone (PCL) are also part of this group (Henkel et al. 2013).
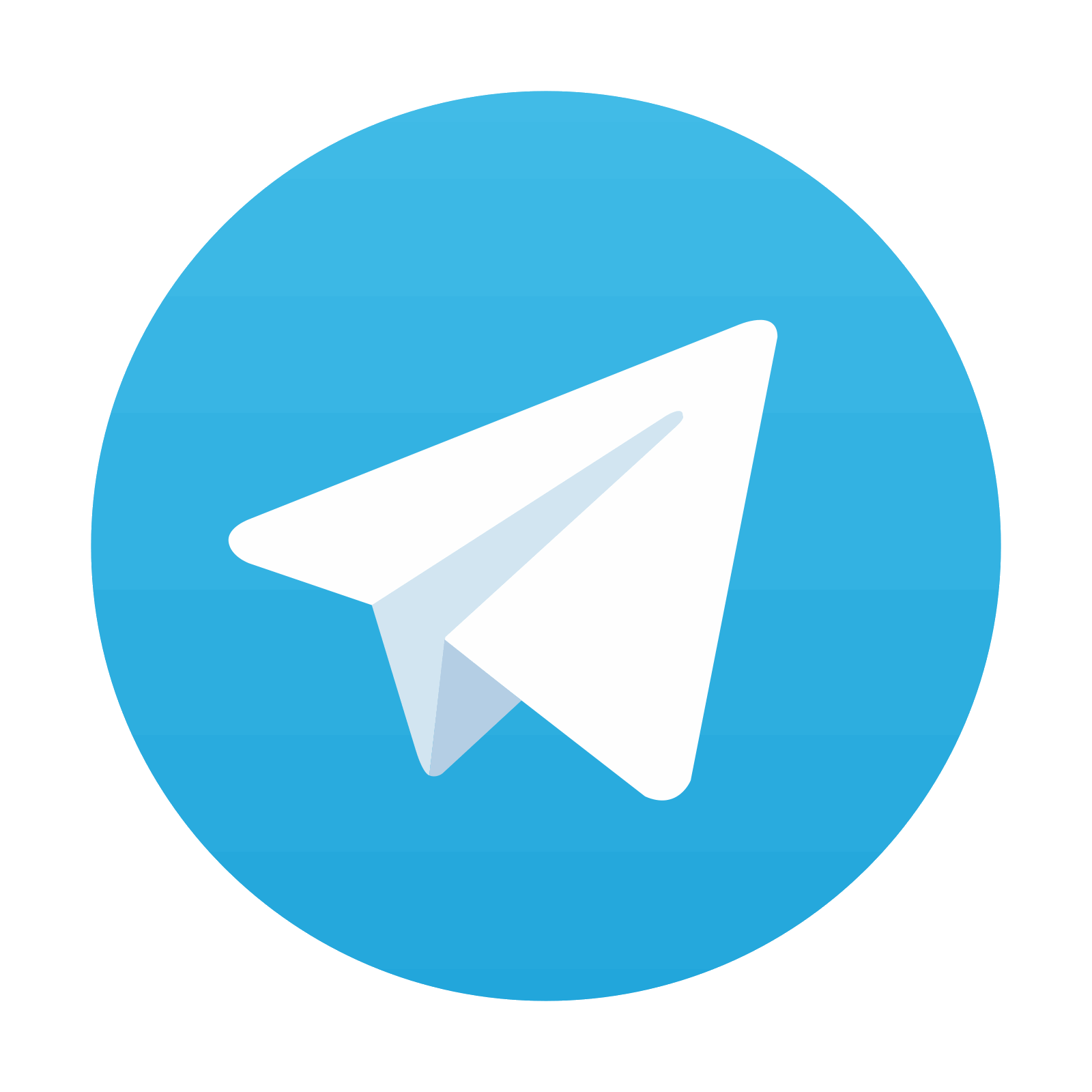
Stay updated, free dental videos. Join our Telegram channel

VIDEdental - Online dental courses
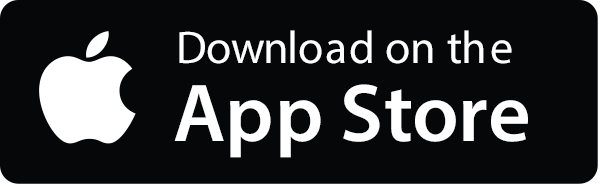
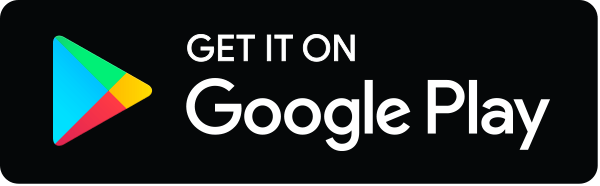