13
Injectable Scaffolds for Oral Tissue Regeneration
Introduction
Regenerative medicine is a field of medicine that has seen a great increase in recent years, this due to the potential it has in repairing or replacing damaged tissues and organs whether caused by trauma, age or illness, as well as congenital defects. The bio-engineering of tissues is a discipline that has been developed during the last 25 years, this discipline, is based on the synergy of three fundamental fields of science; such as cellular engineering, where the cells with the most promising potential due to their capacity for self-replication, their immuno-modulation and, most importantly, their capacity to become any tissues, are stem cells, which have been isolated and characterized by almost any organ; the synthesis of new materials that act as scaffolding or template simulating the physical, chemical and mechanical properties of the native extracellular matrix, these scaffolds are made of three different materials such as: metallic, ceramic and polymeric, being the second factor within bio-technical engineering and finally the addition or functionalization with molecules that induce the biological response of the cells towards the phenotype of the tissue or organ that it is trying to repair or replenish (Chatterjee et al. 2011; Bhattarai et al. 2018).
This chapter focuses on the synthesis of polymeric scaffolds by injection techniques, which simulate the architectural properties of the native extracellular matrix. The techniques described in the literature, such as electrospinning, will be discussed in depth, spinning by propulsion of air to the design of scaffolds by means of 3D and 4D printing. The advantages, disadvantages and challenges of each of these synthesis techniques will be addressed (Ashammakhi et al. 2018; Vazquez-Vazquez et al. 2019).
Electro-spinning
The technique of electro-spinning, is a technique developed in the year 1900, which is an economic technique and more common to develop fibrillar), scaffolds in micrometric and nanometric scales. This technique uses electrostatic forces to deposit polymer solutions, thus forming the said scaffolds.

Figure 13.1. Regenerative medicine, which are confluencing biomolecules, scaffolds and cell engineering.

Figure 13.2. Electro-spinning set up. High voltage supply, metallic collector, polymer solution and pump.
The electro spinning technique consists of a device composed of four key elements: aperistaltic pump containing a syringe with polymer solution, a capillary (needle) to direct the polymer solution into an electro magnetic field, a high-voltage source to eliminate the surface tension of the polymeric solution and a metal collector where the fibers will be deposited, and in this way the nano/micro fibrillary polymeric scaffold is formed (Matthews et al. 2002; Yin et al. 2013).
During the electro-spinning process, the polymer solution stops through the needle, applying an electric potential to eliminate the surface tension and the visco elastic force, resulting in the formation of the Taylor cone. While the solvent evaporates during deposition, the continuous flow of the polymer is collected in a suitable metallic device (Bhattarai et al. 2018). The handling of the parameters of such polymer concentration, the distance between the collector and needle, the speed of deposition and electric field might affect the physico-chemical results in the handling of the properties of the synthetic scaffold. Furthermore, if a rotor coupled to the metal collector is used, it results in obtaining scaffolds with aligned fibers. It is because of these characteristics that the technique of electro spinning, is a simple, flexible and low cost option for the formation of scaffolds that can be applied to different areas of research (Alvarez-Perez et al. 2010; Levorson et al. 2013; Yin et al. 2013). The characterization of the scaffolds are widely reported in literature, such as SEM, TEM, RAMAN and FTIR are the mostly frequently reported.
Air Jet Spinning
Recently, a new technique has been reported, while not completely a method based on a flow of liquid driven by a plunger, it could be considered injectable, since it uses a polymer solution propelled by a flow of air or gas. This technique has been refilled as spinning by air propulsion, in this process with which three-dimensional polymeric scaffolds have been obtained, consists of a device, which is integrated by materials sold in hardware stores; this device has as it’s main feature the use of an air brush connected to a source of air or gas, such as an air compressor or gas cylinder, mainly containing nitrogen or argon and finally a collector that can be of any material lined with aluminum or waxed paper. Like electro-spinning, the properties of the resulting fibrillar scaffolds by means of air pressure spinning vary according to certain parameters such as: polymer concentration, the different types of solvents used for the preparation of the solution, the distance from the tip of the air brush to the collector and the pressure of the air flow used (Medeiros et al. 2009; Abdal-hay et al. 2013).
One of the advantages of the synthesis by means of spinning by propulsion of gas, lies in the simplicity of the device, in how easy it is to obtain the assembly of the said device and how safe it is to operate it in comparison with other techniques that use high voltage for their operation. Among the disadvantages is that in spite of obtaining scaffolds in nanometric and micrometric scales, it is not possible to regulate the fiber diameter size with accuracy, in comparison with the electrospinning technique, in which this is one of its main advantages besides that in the technique of spinning by propulsion of air, only scaffolds with fibers without a defined orientation are obtained, on the other hand with electro-spinning scaffolds can even be obtained with fibers oriented definidamente, which resemble certain characteristics of tissues of the body (Tutak et al. 2013).
Within the biological characterization, it has been reported that mesenchymal stem cells isolated from bone marrow, when placed on these scaffolds and compared with the biological response on scaffolds synthesized by electro-spinning, are similar. It has been reported that in defects in animal models, when implanting these scaffolds, the specimens present inflammation which is possibly due to the concentration of the polymer and also to the non-evaporated solvents, however, after this response the scaffolds show dimensional stability, which is a requirement for tissue regeneration (Granados-Herndndez et al. 2018; Suarez-Franco et al. 2018).
In recent times, a machine has been designed with the same principle of spinning by gas propulsion, this technique has been referred to as a blow spinning solution, in which a semi-automated device, that in addition to integrating the original components of spinning by gas propulsion, has incorporated a steel/aluminum nozzle, especially designed for this purpose, which has the advantage of easy disassembly and cleaning, since in the technique of spinning by air propulsion, it is a disadvantage that increases the concentration of polymer and the time of deposit, by giving rise to variations in the physical properties of the scaffolding, in addition to the nozzle, a mobile base was also incorporated, which allows automated movements in both horizontal and vertical direction and finally a pump injection to control the constant flow of the polymer solution; the other components are the same as the gas propulsion spinning technique. In this way the physical properties such as: fiber diameter size, orientation and no droplet formation are controlled in comparison with gas propulsion spinning; while the chemical properties of the scaffolding are not affected, something that occurs in the same way with electro-spinning and gas-propulsion spinning (Hell et al. 2018).
In dentistry, scaffolds synthesized by gas pressure spinning, the biological response of isolated mesenchymal cells of periodontal ligament has been characterized, where it was found that the response of biological activity such as adhesion and proliferation is related to the diameter of the fibers that in turn depends on the modification of the concentration of the polymer solution, reporting that the lower the polymer concentration, the thinner the fibers which influence the expression of molecules related to the cell adhesion process previously reported on scaffolds synthesized by the electro-spinning technique (Abdal-hay et al. 2013; Suarez-Franco et al. 2018).

Figure 13.3. Air jet spinning set up. Air/gas supply, collector and commercial available air brush.
3D Printing and Bioprinting
Recent advances in tissue engineering owe their success to the development of novel biomaterials-based strategies mimicking native tissues, organ shapes and physiology. These biomaterials are capable of harnessing the innate abilities of cells to sense their local environment through cell—cell and cell—extracellular matrix (ECM) interactions and self assemble into complex networks. Many studies have focused on tuning the bulk properties (i.e., biomolecule concentrations, mechanical properties, cell to cell interactions) of these materials, which assumes that the native cellular environment is homogeneous across multiple length scales. Although modern biomaterials permit the investigation of complex cellular behaviors such as Mesenchymal Stem Cell (MSCs) differentiation and Epithelial-Mesenchymal Transition (EMT) to accurately replicate the heterogeneous nature of native cellular environments. Much of this is due to the inherent difficulty of precisely replicating three dimensional (3D) environments. Although many methods have been developed to generate complex two-dimensional (2D) patterns, biochemical and mechanical cues, 2D culture conditions may not be friendly for cell types. Furthermore, 2D fabrication techniques often cannot be readily translated into 3D culture systems. Therefore, there is a need to adapt these 2D methods and/or create entirely new methods to mimic the complex 3D cellular environment (Liu et al. 2018; Morissette Martin et al. 2019).
Recently, several methods have been developed to spatially encode local properties to 3D materials-based culture systems, and these methods are generically referred to as 3D biofabrication techniques. Such procedures are capable of either constructing or patterning materials, with a high degree of control, by finely tuning and defining material geometries, localization of biomolecular cues, and/or mechanical properties. In doing so, they have created complex material geometries to resemble endogenous tissues. Similarly, biofabrication-based patterning techniques have immobilized controlled concentrations of adhesive ligands, growth factors, or other signaling molecules to mimic cellular architectures in vivo. By enabling this precise control over local and bulk material properties, these methods can create new biomaterials that better replicate the complex and heterogeneous nature of endogenous tissues and organs. This will help to elucidate the gap between the state of tissue engineering and the unrealized hope of true artificial organs and tissues. In addition, this level of control could allow for a more complete model of cancerous or diseased states in cells and tissues to assess current or develop new, therapeutic strategies (Bajaj et al. 2014).

Figure 13.4. 3D biopnnt machine for polymeric intelligent biomaterials.
4D Printing and Bioprinting
Four dimensional (4D) printing was first introduced in 2013 and immediately spurred great attention in various research areas including, but not limited to, smart materials and biomedical research (Yang et al. 2019). Most 4D structures are developed by incorporating shape transformation within a material/structural design, which contributes to the 4D definition: 3D printing of objects which can, immediately after printing, self-transform in function or form when exposed to a predetermined stimulus, including osmotic pressure, exposure to heat, current, ultraviolet light or other energy sources. Shape memory materials have the inherent capacity to fix a temporary shape and recover their permanent structure under suitable stimuli, which is extremely similar in principle with the 4D dynamic process. 3D printing of shape memory materials is reported as novel 4D printing in recent articles; this is becoming a unique and rapidly expanding, research area in 4D printing. One argument in this field is whether controlled degradation of 3D printed constructs can be classified as a 4D effect, a claim noted in some articles. 3D printing has demonstrated great potential in biomedical fields. If biodegradation is included as a tunable mechanism of incorporating a time-dependent effect, 3D printing of timed-release therapeutics and other biodegradable structures fall under 4D printing. A point of critique in this argument is that the 3D printed structures noted above completely disappear in the dynamic processes. On the contrary, in shape or functional transformation through the 4D process, most of the 3D printed structures remain intact; that is, the 3D fabricated structures are the carrier of the shape or functionality shift as a product of the material properties of the print medium. As such, degradation of 3D printed constructs will not be treated as a 4D effect in this context. Although the surrounding environments may change significantly during the 4D process, the configuration or function before and after the stimulation should be structurally or functionally stable. For example, if a 3D fabricated construct has a primary conformation ‘A’ in air and changes to conformation ‘B’ in water, both conformations should be structurally stable, without external forces to maintain its structure (Ashammakhi et al. 2018; Yang et al. 2019).
Based on the concept of 4D printing, the fabricated structure performs a self-assembly process immediately after 3D printing; this self-assembly is a process in which a pre-existing form dynamically changes to another structure as a consequence of external stimuli. Actually, 3D printing of self-assembly structures existed prior to the definition of 4D printing. For example, shape-changing architectures were inkjet printed by patterning light-absorbing ink onto a prestrained polystyrene substrate; the ink acted like hinges to induce an autonomous shape change while they transferred the absorbed heat to the underlying substrate. Additionally, 3D aqueous droplets, with a volume of — 65 pL, were ejected into a lipid containing oil bath and programmed into pre-designed, bilayered networks which swelled or shrank in response to water flow, resulting from osmotic-pressure differences; a variety of shapes were fabricated through the use of droplet networks. 4D printing, however, is leading to greater attention, in various research fields, after the definition was coined (Miao et al. 2017; Morissette Martin et al. 2019).
The repair and regeneration of craniofacial tissues continue to be a challenge for clinicians and biomedical engineers. Reconstruction of pathologically damaged craniofacial tissues is often required because of tumors, traumas, or congenital malformations. There are constructive procedures for craniofacial tissue regeneration which are usually complex because the craniofacial region is a complex construct, consisting of bone, cartilage, soft tissue and neurovascular bundles. For instance, to reconstruct damaged craniofacial bones, surgical procedures are available. Autologous bone grafts have been considered the reference standard for bone regenerative therapies. Together with allogenic bone grafts, this type of bone graft material comprises more than 90% of grafts performed. However, these grafting procedures have numerous disadvantages, including hematomas, donor site morbidity, inflammation, infection and high cost (Morissette Martin et al. 2019).
However, the biomechanical properties of the tissues regenerated through these treatment options are mediocre compared with those of native articular cartilage. Furthermore, the repair and regeneration of muscle tissue (for example, tongue muscle) after traumatic injuries frequently exhibit a challenging clinical situation in the craniofacial region. Substantial esthetic and functional issues will arise if a significant amount of tissue is lost, because of the inability of the native muscle tissue to regrow and fill the defect site. To find an alternative treatment option for the reconstruction of craniofacial tissue, clinicians and scientists have been analyzing new approaches in craniofacial tissue regeneration to maximize patient benefit and minimize related complications. Craniofacial tissue regeneration using Mesenchymal Stem Cells (MSCs) presents an advantageous alternative therapeutic option. MSCs are multipotent cells that are capable of multiple lineage differentiation based on the presence of inductive signals from the microenvironment. MSCs reside in a wide spectrum of postnatal tissue types and have been successfully isolated from several orofacial tissues. Studies have confirmed the self-renewal and multilineage differentiation capacities of orofacial-derived MSCs and have shown that they have better growth properties than Bone Marrow Mesenchymal Stem Cells (BMNISCs). Therefore, dental MSCs are attractive for craniofacial applications as they may be better at differentiating into craniofacial tissues.
Biomaterials are widely used to engineer the physiochemical properties of the extracellular cell microenvironment to tailor niche characteristics and direct cell phenotype and differentiation. Such interactions between stem cells and biomaterials have largely been studied by introducing the cells into 2- or 3-dimensional scaffolds, or by encapsulating cells within hydrogel bio-materials. Alginate hydrogel has been used extensively as a vehicle for stem cell delivery in tissue regeneration. The ability to control the spatial presentation of alginate enables the examination of the effects of alginate hydrogel on stem cell differentiation in a systematic way. In the current chapter, the application of dental-derived MS Cs and alginate hydrogel for potential applications in craniofacial tissue regeneration is emphasized (Yang et al. 2019).

Figure 13.5. Differences among 3D and 4D resulting printing.
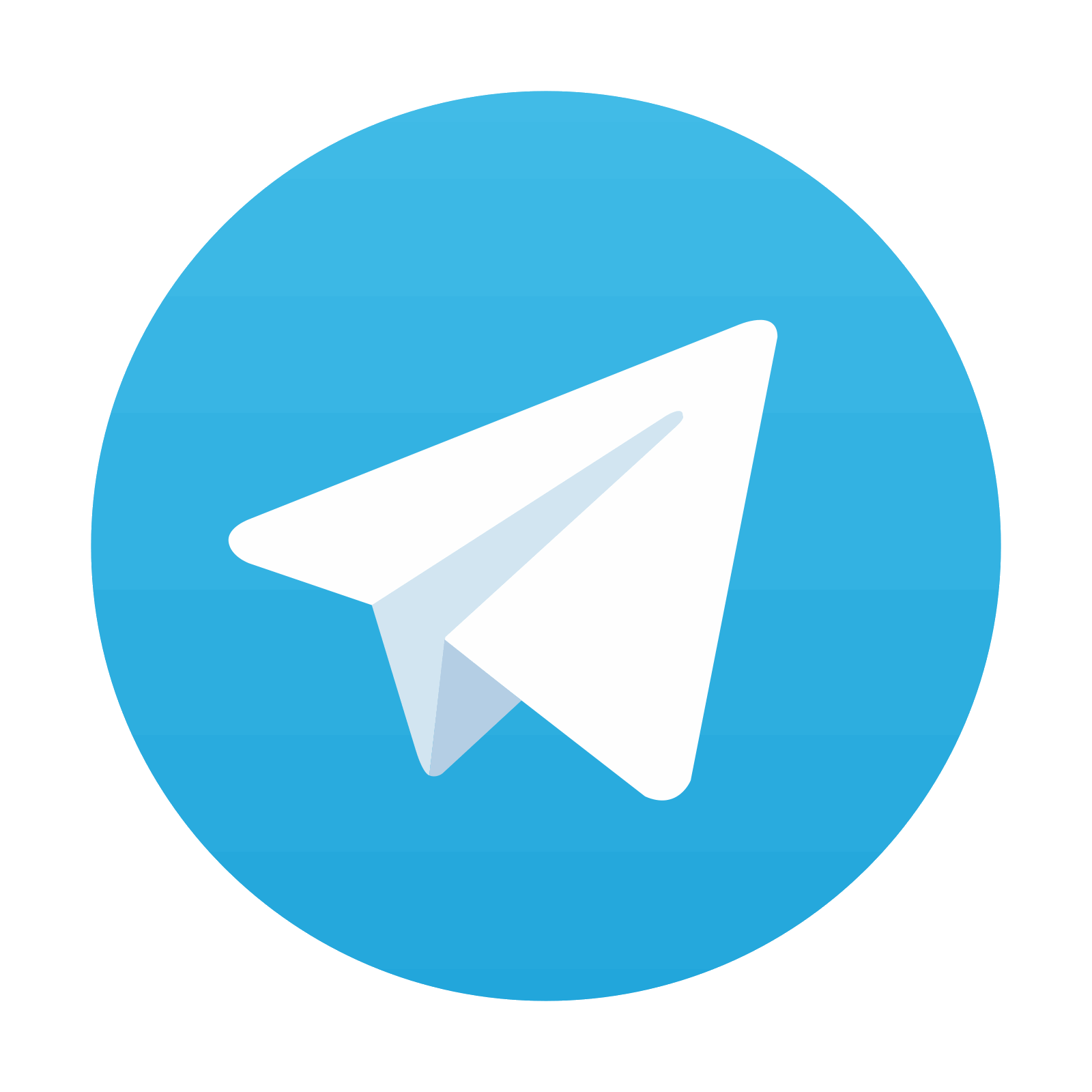
Stay updated, free dental videos. Join our Telegram channel

VIDEdental - Online dental courses
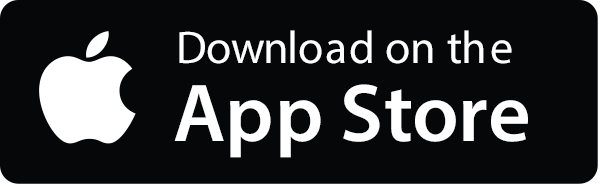
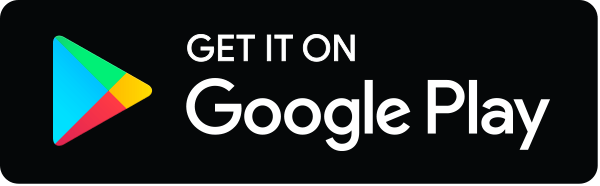