7
Composite Materials for Oral and Craniofacial Repair or Regeneration
Introduction
Over the past decade, a wide range of degradable, partially degradable and non-degradable polymer based composites has been investigated to repair or to regenerate hard tissues in oral and craniofacial surgery. These composites can be prepared in the laboratory and then implanted or they can be polymerized in situ. For the former approach, the main advancements arise from Additive Manufacturing (AM) technologies, also known as 3D printing, while injectable or spreadable nanocomposites represent the main achievements for in situ forming of prostheses, restorative materials and scaffolds for Tissue Engineering (’l’E). Self-shape adaptation of injectable or spreadable nanocomposites, and the overall reduced time from diagnosis to implantation, is the most convenient approach for dental and cranial bone tissues repair or regeneration. However, several drawbacks such as heat and shrinkage due to the polymerization process and release of unreacted monomers, limit the in situ forming approach. On the other hand, patient tailored prostheses and scaffolds, designed and manufactured in the laboratory, involve the use of the Reverse Engineering (RE) applied to organs and hard tissues for defining, via Computer Aided Design (CAD), the customized prosthesis or scaffold. 3D imaging clinical tools like X-ray CT, MRI and Laser scanners provide the main data source for developing the digital model. Implant designing, composite materials and engineering technologies, as well as future trends in the field, will be focused.
Surface scanners provide the 3D point cloud representing anatomical features which undergoes an editing process involving: filtering and cleaning of the 3D point cloud data-set (Fig. 7. 1a), slicing according to a determined direction (Fig. 7.1b), definition of oriented parametric curves (Fig. 7. 1c), and definition of parametric surfaces and mesh (Fig. 7. 1d). Avariety of software has been developed to edit the 3D point cloud (e.g., RapidForm) (Da Silveira et al. 2003; De Santis et al. 2018).
Volume scanners (e.g., X-ray CT) data undergoes a segmentation process to distinguish the variety of tissues constituting the organ: mandible ramous cross section (Fig. 7.1e), mandible arch cross section (Fig. 7.1f), condyle cross section (Fig. 7.1g), 3D mandible model (Fig. 7.1h). Avariety of software has been developed to edit and process volume data (e.g., Mimics) (Dean et al. 2003; De Santis et al. 2005). The outer surface of anatomical features is often described by an STL format, which is also the common file format in AM.

Figure 7.1. Surface scanning of the skull: Cleaned and filtered 3D point cloud data-set (a), slicing (b), definition of parametric curves (c), definition of parametric surfaces and mesh (d), volume scanning of the mandible: mandible ramous cross section (e), mandible arch cross section (f), condyle cross section (g), 3D mandible model (h).
Cranioplasty
Cranioplasty is a common procedure for correcting bone defects or deformities in the cranium arising from traumatic skull bone fracture, cranial bone deformities and bone cancer and infections. Surgery involving the use of biocompatible materials, and repair or regeneration of large cranial defects is particularly challenging from both the functional and aesthetic point of view (De Santis et al. 2010; Piitulainen et al. 2015; Unterhofer et al. 2017).
At least four approaches involving the use of polymers and composites can be categorized. The first and simplest approach is the in situ application of the biomaterial (Costantino et al. 1993; Lee et al. 2009). The second approach is ex vivo and considers a plaster impression taken over the defect for realizing a mould into which the prostheses or scaffold is formed (Caro-Osorio et al. 2013; Jaberi et al. 2013). The third ex vivo approach uses a 3D scan of the defect for realizing, through AM, a mould into which the prostheses is formed (Saringer et al. 2002; Peltola et al. 2012; Msallem et al. 2017). The fourth approach uses 3D scanning of the defect and RE in conjunction with AM to directly manufacture the prosthesis or scaffold (Espalin et al. 2010; Han et al. 2019).
3D surface imaging through laser scanners and photogrammetry are gaining popularity in this filed as these approaches are less invasive than other clinical imaging tools such as X-ray CT (Arridge et al. 1985; Mertens et al. 2017).
Polymer based Composite Materials in Cranioplasty
Mechanical performances play a crucial role in the design of composite scaffolds for hard ‘l’E applications: hard tissues such as bone are stiffer (higher elastic modulus) and stronger (higher strength) than soft tissues. In this context, polymer-based composite materials provide an alternative choice to overcome the problems related to the flexibility and weakness of many polymers. Several criteria have to be considered in designing composite materials. In particular, the stress transfer from polymeric matrix to the reinforcement plays a pivotal role in enhancing composite strength, whilst the different ductility between the polymeric matrix and the reinforcement should create discontinuities in the stress transfer and the generation of stress concentration at the particle/matrix interface.
Poly-Methyl-Methacrylate
Poly-Methyl-Methacrylate (PMMA) is the polymer most widely used for cranioplasty. Its history is longer than half a century and in some instance it showed better long-term outcomes compared to frozen autologous bone (Moreira-Gonzalez et al. 2003; Piitulainen et al. 2015). PMMA can be adopted according to all the four approaches listed earlier. For the in situ forming approach, PMMA is provided to surgeons in the form of a solid powder phase made of PMMA and/or copolymers and a liquid monomer component. By mixing the powder and liquid phases the paste is applied in vivo and a radical polymerization reaction occurs driven by benzoyl-peroxide and amines (i.e., activators/co-initiators for the formation of radicals) contained in the powder and liquid phase, respectively (Harris et al. 2014; De Santis et al. 2003). Once polymerized, mechanical properties (Table 7.1) of PMMA are in between those of the spongy and cortical bone (McElhaney et al. 1970; Ronca et al. 2014). Heat developed during the exothermic reaction of PMMA limits the direct intra-operative use of this bone cement, especially if large cranial defects need to be restored. However, PMMA can be easily processed through the moulding strategies (van Putten et al. 1992; Cheng et al. 2018; Maricevich et al. 2019). The main advancement of PMMA relies on modifications of the acrylic cement to improving biological and mechanical functions. The loading of antibiotics (i.e., gentamicin) is suggested to prevent infection (Minelli et al. 2011; Worm et al. 2016). However, a slight reduction of mechanical properties for gentamicin loaded PMMA has been observed (De Santis et al. 2003). The realization of PMMA based nano-composites has been suggested for antimicrobial purposes by incorporating silver (Oei et al. 2012) and gold (Russo et al. 2017) nanoparticles into the polymeric matrix. The integration of phase-change particles has been suggested to reduce temperature levels during polymerization (De Santis et al. 2006). Several PMMA based composites for cranioplasty have been advised for improving osteoconductivity and biocompatibility. Hydroxyapatite (Itokawa et al. 2007; Zebarjad et al. 2011) and bioactive glass (Peltola et al. 2012) represent the most common type of particles functionalizing and reinforcing the acrylic matrix (Table 7.1). Medical-grade PMMA filaments represent the future trends in cranioplasty through AM (Espalin et al. 2010), and gentamicin doped filaments have also been investigated (Mills et al. 2018).
Table 7.1. Young’s modulus of polymer based composite materials.
Composite material | Testing condition | Young’s modulus [GPa] | Reference |
---|---|---|---|
Cortical/trabecular bone from the skull | Compression | 2.41–5.58 | McElhaney et al. 1970 |
Cortical/trabecular bone from the macaca mulatta | Compression | 6.47 | |
Trabecular bone from tibia | Bending | 0.05-0.3 | Ronca et al. 2014 |
PMMA (Symplex-P) | Bending | 2.6 | De Santis et al. 2003 |
PMMA (CMW1/Gentamicin) | Bending | 2.3 | |
PMMA/HA | Bending | 2.0 | Zebarjad et al. 2011 |
PMMA/AgNP | Bending | 1.7–2.4 | Oei et al. 2012 |
PEEK 450G | Bending | 3.8 | Arif et al. 2018 |
PEEK 450G | Tensile | 4.0 | |
PEI | Bending | 9.1 | De Santis et al. 2000 |
PEI | Tensile | 14.3 | |
PEEK/PEI/TiO2 | Tensile | 5.3 | Diez-Pascual and Diez-Vincente 2015 |
C-FRP-PEEK | Tensile | 7.4 | Han et al. 2019 |
G-FRP-PEI | Bending | 10.9 | De Santis et al. 2000 |
C-FRP-PEI | Bending | 57.7 | |
PCL | Compression | 0.60 | Wang et al. 2018 |
PCL | Tensile | 0.59 | De Santis et al. 2011 |
PCL/FeHA | Tensile | 0.68 | |
PLA | Tensile | 1.5 | Mi et al. 2013 |
PLA | Compression | 0.63 | |
PGA | Tensile | 6.9 | Yang et al. 2001 |
PLGA | Tensile | 1.4–2.8 |
Poly-Ether-Ether-Ketone and Poly-Ether-Imide
A growing interest in alloplastic cranioplasty through high-performance polymers, such as Poly-Ether-Ether-Ketone (PEEK) and Poly-Ether-Imide (PEI), is also recognized PEEK and PEI are considered expensive thermoplastic semicrystalline polymer showing outstanding mechanical (Table 7.1) and chemical resistance (Arif et al. 2018; Cicala et al. 2018). PEEK cranioplasty is radiolucent and can be sterilized by steam or gamma irradiation (Lethaus et al. 2012; Shah et al. 2014). Short-term follow-up indicates that PEEK is a material of choice especially for patients showing a premorbid neurological status (Lethaus et al. 2011). A large patient series receiving a PEEK temporo-parietal region reconstruction support the high potential of this material for large cranio-facial defects (Ng and Nawaz 2014). A recent ten-year follow up suggests that PEEK cranioplasty leads to a normal progressive healing similar to titanium mesh (Piitulainen et al. 2015). Medical-grade PEEK filaments represent the future trends in cranioplasty through cutting edge technologies of AM (Honigmann et al. 2018), and carbon fibre reinforced PEEK filaments provide further mechanical enhancement (Han et al. 2019). On the other hand, PEI is a well-known polymer in the biomedical field for its biocompatibility (Richardson et al. 1993; Merolli et al. 1999), excellent mechanical properties (De Santis et al. 2000; Cicala et al. 2018) chemical and thermal stability (Johnson and Burlhis 1983). The aforementioned excellent properties, together with the easy sterilization capability (Eltorai et al. 2015), suggested PEI for the manufacturing of surgical guides for cranioplasty (Bekeny et al. 2013; Yang et al. 2018). PEI composites reinforced with continuous carbon and glass fibres have been proposed for the realization of advanced composite bone prostheses (De Santis et al. 2019; Gloria et al. 2008). PEI based composites, incorporating 4%ww titanium dioxide nanoparticles, have further shown antibacterial action versus Gram-positive and Gram-negative (Diez-Pascual et al. 2015). In the last decade, filaments of PEI and PEI blends (also known as ULTEM), are gaining attention for AM processes, representing the future trends in orthopaedics (Parthasarathy 2015; Lee et al. 2017).
Glass or Carbon Fibre Reinforced Composites
Bioactive composite sandwich, consisting of Glass or Carbon Fibre Reinforced Composites (G-FRC or C-FRC) laminates and a core of Bioactive Glass (BG) particles, have also been considered for cranioplasty. G-FRC based on a methacrylic resin matrix coated with BG granules has shown the potential to promote the healing process of calvarial bone defects in rabbits (Tuusa et al. 2008). Soon after, G-FRC based on a dimethacrylic resin matrix incorporating BG granules was used, on humans, for a complex clinical case of a patient undergoing repetitive cranioplasty. The G-FRC/BG explant (after 27 months) showed osteoid formation together with small clusters of mature hard tissue being observed at the margin of the cranioplasty, suggesting that this composite represents a feasible method for calvarial reconstruction (Posti et al. 2016). A G-FRC/BG cranioplasty, based on a photopolymerized dimethacrylic resin matrix and a highly porous BG structure, has also shown antimicrobial and osteoconductivity features (Aitasalo et al. 2014). The advantage of using a photo-cured G-FRC laminate sandwich in conjunction with a BG core is that the material can be precisely layered before polymerization occurs and high load-bearing capability can be expected if the external composite laminates are properly spaced (Piitulainen et al. 2017). A three-year follow-up investigation on paediatric patients with a large cranial defect restored with a thin G-FRC laminate and a core BG scaffold concluded that this composite is a safe and a functional solution for restoring cranial defects in paediatric populations (Piitulainen et al. 2015). C-FRP medical grade implants consist of a woven carbon fabric impregnated with a biocompatible thermoset epoxy matrix. Each C-FRP lamina is laid-up on the positive templates of the cranial defect, and a 3 years follow-up on 27 patients has shown no postoperative complications (Saringer et al. 2002). Although some complications such as infection and adverse reactions may occur, excellent cranioplasty restorations through C-FRP have been observed on a wider sample (Wurm et al. 2004).

Figure 7.2. . RE approach in cranioplasty and AM model and scaffolds. 3D imaging through TAC (a), CAD model (b), IJP skull solid model (c), SLA scaffold model (d), FDM scaffold model (e).
Degradable Polymer based Scaffolds
Degradable scaffolds for cranial TE (Hassan et al. 2019) are particularly important for paediatric patients whose skull growth adds an additional concern in the selection of non-degradable material. Poly-Lactic-Acid (PLA) outer membranes and a HA cement inner core represent a first example for regenerating skull bone defect (Cohen et al. 2004). Tensile and compressive Young’s modulus (Table 7.1) of PLA are in between those of a trabecular and cortical bone (Mi et al. 2013). A fully degradable cranioplasty has been achieved following the same strategy but using ß-tricalcium phosphate granules enclosed between custom-moulded PLGA mesh (Thesleff et al. 2017). Poly-Lactic-co-Glycolic-Acid (PLGA) membranes have also been used in conjunction with autologous bone according to the in situ approach to regenerate skull deformities, suggesting that this approach is safe with tolerable morbidity rates in children (Gephart et al. 2013). Following a similar approach, a PLGA mesh has been layered in vivo onto on the dura, a demineralized bone matrix is applied onto the bio-degradable mesh, and a second PLGA mesh is layered to form a sandwich like structure (Chao et al. 2009). This cranioplasty technique is useful if the autologous bone is not available, and it has also promoted bone regeneration with no morbidity. Another interesting approach for cranial bone regeneration has used PLGA meshes contained in the inner core ß-tricalcium phosphate granules in conjunction with autologous adipose derived stem cells (Thesleff et al. 2017). PLGA scaffolds with a morphologically controlled micro-architecture, realized through FDM, have been implanted in the parietal skull defect in mice model; with three weeks of in vivo observations, angiogenesis originating from desmal bone was evident (Sinikovic et al. 2011). PLGA micro-sphere incorporating bone morphogenetic proteins further enhanced the capability of ‘l’E to regenerate cranial bone tissue (Wink et al. 2014). Young’s modulus of PGA is close to that of the cortical bone (Table 7.1), while the modulus of PLGA can be tailored over a wide range according to the copolymer composition (Yang et al. 2001). Poly-Capro-Lactone (PCL) is another interesting degradable polyester used for the manufacture of morphologically controlled and full interconnected architectures for bone regeneration (Russo et al. 2013). This polymer has mechanical properties similar to a dense spongy bone (Table 7.1) and its low melting point allows an easy process through AM (De Santis et al. 2011).
Nowadays, the possibility to obtain scaffolds for the repair of skeletal defects in the field of tissue engineering should present a viable alternative to conventional treatment (Hutmacher 2000).
An ideal scaffold should promote bone regeneration and also target the process to certain regions. In particular, it should be advantageous for surgical correction of craniosynostosis in encouraging the formation of cranial bone tissues, while preventing resynostosis (Fedore et al. 2017). Furthermore, medical imaging data would allow for precise and customized replication of the architecture of the skull and bony defect, in a combined approach of RE and AM (Chim and Schantz 2005), thus simplifying surgical placement and retention.
With the latest advancements of 3D scanning, design software and printing technologies, 3D printing of individually customized tissues scaffolds can be created for clinical use (Morrison et al. 2015).
In this scenario, the best combination of materials properties and technologies advancement still represents a challenge in 3D printing research for in vivo applications.
PCL scaffolds, manufactured through AM and seeded with mesenchymal progenitor cells and calvarial osteoblasts, have been tested in critical-size defects of the calvarial rabbit model, and results suggest that these 3D constructs have the potential for bone regeneration (Schantz et al. 2003). Short-term human clinical trial suggests that PCL scaffolds realized through AM and coated with bone marrow are well tolerated, have excellent biocompatibility, act as a bone guiding template, and can be firmly anchored to the hosting calvarial tissue (Schantz et al. 2006). A custom-made PCL scaffolds that allow for calvarial osteoblast differentiation and mineralization in vitro can be utilized to prevent osteoblast differentiation if coated with polyethylene glycol hydrogel (PEG hydrogel), thus providing a specific control in bone formation during tissue regeneration (Fedore et al. 2017). PCL composites, incorporating calcium phosphate at a weight ratio 1:1, have been implanted in the calvaria of a sheep model, and have shown desirable osteoconductivity and osteointegration (Wang et al. 2018).
A dynamic seeding of osteoblasts and endothelial cells onto a 3D fibre-deposited PCL scaffold may represent a useful approach in order to achieve a functional hybrid in which angiogenesis, furnished by neo-vascular organization of endothelial cells, may further support osteoblasts growth (Kyriakidou et al. 2008).
However, the poor cytocompatibility of the synthetic polymers leads to the inefficiency of the scaffold in obtaining a friendly interface with living cells. Consequently, in order to improve the cell guidance ability of synthetic PCL-based scaffolds (Fig. 7.3a), different modifications have been proposed, the aim being to influence surface properties (i.e., surface charge, wettability, roughness and topography). Surface treatment such as y-ray irradiation, plasma treatment, endgrafting, ozone oxidization or in situ polymerization have been proposed to modify the materials surface properties. However the possibility to introduce amino groups onto the polyester surface through a reaction with diamine will result in decreasing of surface hydrophobicity, neutralization of the acid originated from the scaffold degradation, and the possibility to provide active sites through which other biomolecules such as gelatin, collagen or arginine-glycine-aspartic acid (Arg-Gly-Asp or RGD) peptides can be immobilized. PCL containing laminin-derived peptides sequences IKVAV (Ile-Lys-Val-Ala-Va), YIGSR (Tyr-Ile-Gly-Ser-Arg), or integrin-derived peptides sequence RGD, covalently linked to the polymer surface evidenced an enhancement in cell adhesion and spreading (Santiago et al. 2006; Causa et al. 2010; Gloria et al. 2012).

Figure 7.3. Polyester based composite scaffolds. SEM image of PCL scaffold obtained through FDM and seeded with hMSC (a), Atomic force microscopy showing the topography of neat PCL (b), SEM image of PEG/HA nanocomposite scaffold obtained through SLA (c), Atomic force microscopy showing the topography of PEG/HA (d).
The conjugation of amine-terminated peptides by means of reductive amination after tether insertion on 3D well-organized scaffolds obtained through AM approach has shown a specific recognition of the solid signal to NIH3T3 integrin cell receptors suggesting a correct presentation of the peptide sequences, taking into consideration its effect on their macromechanical behaviour.
Composite scaffolds based on a PCL matrix have also been investigated for bone TE.
To overcome the limitation of cell penetration and osteoconductivity improvement of the PCL-based implants for craniofacial tissue engineering, composite scaffold of polyvinyl alcohol (PVA), PCL and Bioceramic (HAB) have been proposed. PVA has been adopted since it introduces several free hydroxyl chains, which can be used for scaffold functionalization via linking drugs, biomolecules, or growth factors (Orienti et al. 2001; Prabha et al. 2018), whilst HAB represents a triphasic bioceranvic developed by incorporation of hydroxyapatite, beta tricalcium phosphate, calcium silicates and traces of magnesium synergistically acting in improving to produce an osteoconductivity and osteoinductivity (Jones 2013; Prabha et al. 2018). Results indicate that these composite structures should support attachment and growth of stromal stem cells (human bone marrow skeletal (mesenchymal) stem cells (hMSC) and Dental Pulp Stem Cells (DPSC)). In addition, the scaffold supported in vitro osteogenic differentiation and in vivo vascularized bone formation.
Bioactive PCL and titanium as well as PCL and zirconium organic—inorganic materials have been suitably synthesized by the sol-gel method, highlighting that these organic—inorganic hybrid materials exhibit a hydroxyapatite layer on the surfaces of samples soaked in a fluid simulating the composition of human blood plasma (Russo et al. 2010).
Mechanical performances play a crucial role in the design of composite scaffolds for hard tissue engineering applications: hard tissues such as bone are stiffer (higher elastic modulus) and stronger (higher strength) than the soft tissues. In this context, polymer-based composite materials provide an alternative choice to overcome the problems related to the flexibility and weakness of neat polymers (i.e., PCL). Several criteria have to be considered in designing composite materials. In particular, the stress transfer from polymeric matrix to the reinforcement plays a pivotal role in enhancing composite strength, whilst the different ductility between the polymeric matrix and the reinforcement should create discontinuities in the stress transfer and the generation of stress concentration at the particle/matrix interface.
Thus, composite materials based on PCL matrix reinforced with PCL/TiO2 or PCL/ZrO2 hybrid fillers should enhance the mechanical performance of the neat PCL and, at the same time, should improve their bioactivity and biological performances (Russo et al. 2010).
In the innovative concept of magnetic cell guidance, nano-composite polyester substrates incorporating magnetic nanoparticles (MNPs) or iron doped nano-HA particles (Fig. 7.3c) has been also analyzed (Gloria et al. 2013). MNPs may be heated up, allowing their use as hyperthermia agents able to deliver thermal energy to targeted bodies (i.e., tumours) or as elements capable of improving chemotherapy or radiotherapy by providing a degree of tissue warming appropriate for the destruction of malignant cells. The idea of manipulating and controlling specific processes at the cell level by releasing biomolecules and bioactive factors, in turn, linked to magnetic nanocarriers has been proposed. Controlled differentiation of human bone marrow stromal cells using remote magnetic field activation and MNPs has been proposed (Kanczler et al. 2010).
The idea to develop magnetic scaffolds for additionally controlling angiogenesis in vivo was first considered by (Bock et al. 2010).
A magnetic scaffold should modify the external magnetic flux distribution, thus causing a much higher concentration of magnetic flux in the vicinity of and inside the scaffold (Gloria et al. 2013). In this context, enhanced cell-material interaction, at least in vitro have been proved when PCL scaffolds were reinforced by the inclusion of iron-doped hydroxyapatite (Gloria et al. 2013), while they have promoted, in vivo on a rabbit model, new bone formation after 4 weeks from implantation (De Santis et al. 2015a).
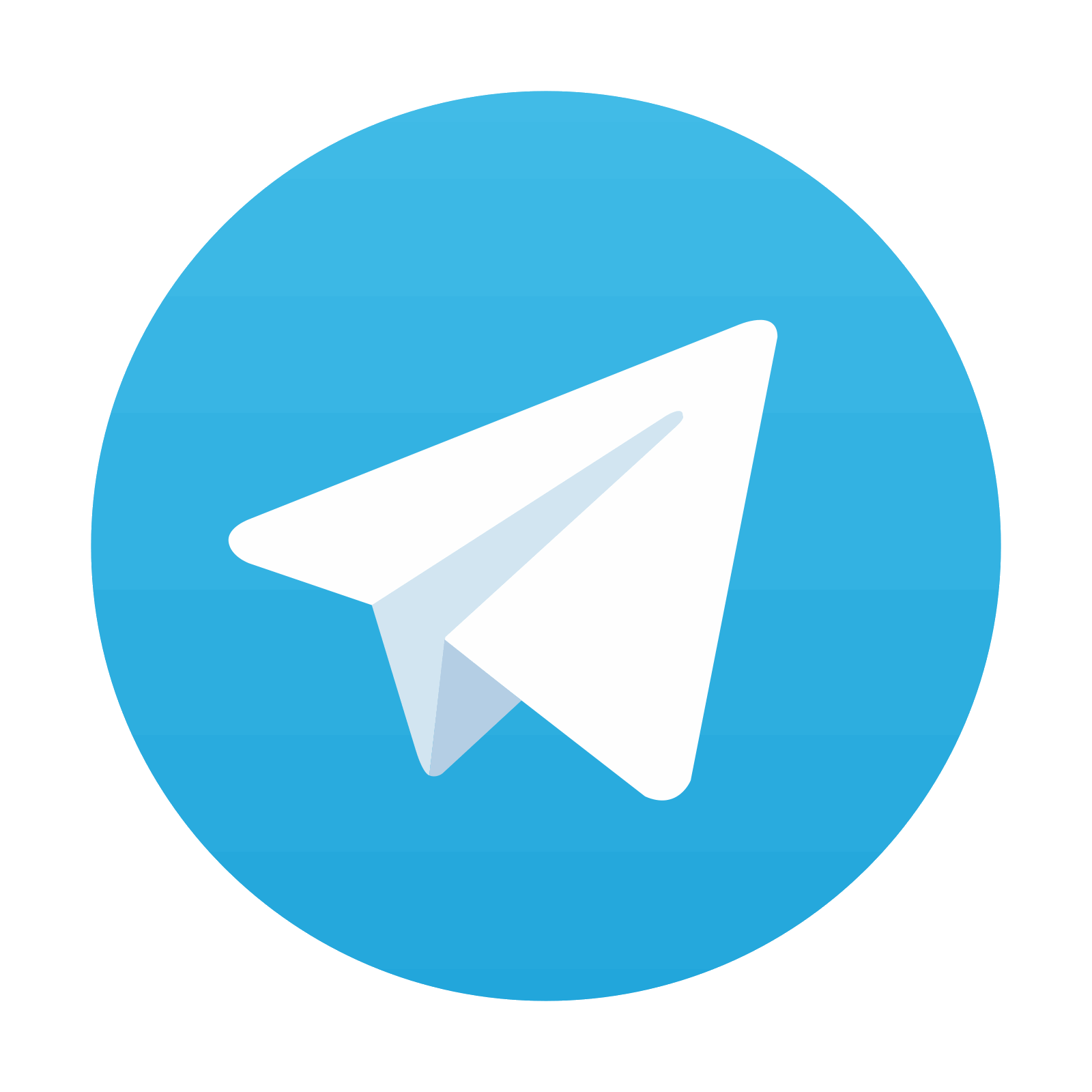
Stay updated, free dental videos. Join our Telegram channel

VIDEdental - Online dental courses
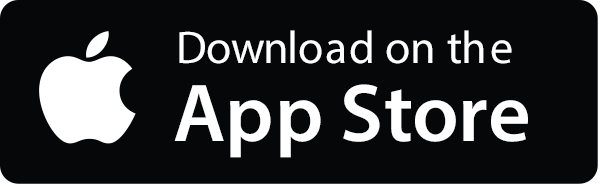
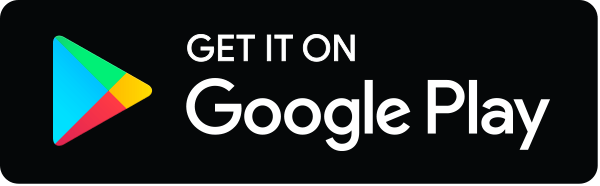