14
Clinical Applications of Digital Dental Technology in Maxillofacial Prosthodontics
Muhanad Moh’d Hatamleh
14.1 Introduction
Maxillofacial prosthodontics is a subspecialty of prosthodontics that rehabilitates patients with maxillofacial defects by means of removable maxillofacial prostheses. Such defects are usually acquired due to trauma or surgical excision or are of congenital origin (Hatamleh et al. 2010). The prosthesis aims to restore patient’s aesthetics, appearance, and function, which ultimately enhance their self‐esteem and reintegration into society toward an improved quality of life. A prosthesis of suboptimal aesthetics can be disfiguring to the patient as it attracts attention and is usually abandoned. Hence, an ideal prosthesis should restore the missing anatomical contours accurately, be of good camouflage of the defect, and be color‐matched to the tissues surrounding the defect (Phasuk and Haug 2018, Hatamleh et al. 2021).
Maxillofacial prostheses are traditionally manufactured following several lengthy procedures that can be traumatic for the patient and rely on the skills of the individual manufacturing team who could be an anaplastologist, maxillofacial prosthetist, or clinical reconstructive scientist (Hatamleh et al. 2010). Digital technologies have gained increased interest in different fields of dentistry (Michelinakis et al. 2021), but their integration in maxillofacial prosthodontics practice is still slowly developing (Cristache et al. 2021, Wolfaardt et al. 2021).
Advancement in body scanners whether at the computed tomography (CT) level or surface scanners meant that with the aid of specialized medical software, quality prosthetics can be successfully maintained. Advanced specialized software enable the reconstruction of patient digital scan into three‐dimensional (3D) visualization, and virtually reconstruct the missing part, which is then digitally reproduced in biocompatible materials using 3D printers. This digital/technical workflow enabled optimized prosthetics along with reducing individual subjectivity incurred in the past (Peng et al. 2015, Nuseir et al. 2019, Cristache et al. 2021).
Such digital technologies were considered central to the future of maxillofacial prosthetics and important to attract younger colleagues to the subspecialty. A UK‐based survey study showed that one‐third of maxillofacial units use digital technologies in the form of impression‐taking, prosthesis‐planning, and skin color reproduction in their maxillofacial prosthetics practice (Hatamleh et al. 2010). They emphasized the need to expand their use toward complete integration into services. In North America, maxillofacial prosthodontists perceived changes attributed to surgery and advanced digital technologies as the key changes taking place in care delivery in the past 10 years; yet the majority of them believed their training programs were not providing adequate education and training in the use of advanced digital technology in maxillofacial rehabilitation (Wolfaardt et al. 2021).
Digital technologies have enhanced data acquisition and prosthesis manufacturing capabilities. This chapter will focus on the current status of digital technology integration in the fabrication of extraoral maxillofacial prosthetics.
14.1.1 Conventional Maxillofacial Prosthetics Workflow
Conventional construction of maxillofacial prostheses involves mainly three phases: preparation, production, and placement phases and requires patient presence in most of them (Naveen 2018). The first two phases include several complex steps and rely on the artistic ability, skills, and subjectivity of the anaplastologists, maxillofacial prosthetist, or technician involved.
The preparation phase involves reproduction of area requiring a prosthesis by means of impression‐taking and casting toward providing a positive mold of the defect upon which a wax or clay prototype is produced to restore missing anatomies. The prototype is usually enhanced by fine morphological carving and contouring, surface‐texturing, and detailing toward almost‐perfect resemblance to the existing contralateral side. Patient pre‐defect reference photos, molds, and/or defect contralateral structures (i.e. ear, orbit) are usually cited during the manual prototype production with or without patient presence. The prototype is optimized in shape, contour, and fit by the maxillofacial prosthetist’s subjective view and over separate multiple appointments. This can be tiring to the patient and maxillofacial prosthetist alike. The wax prototype is then flasked by a manual mixing of dental stone and molding. The mold is heated and the molten wax is removed in preparation to pack the mold with a silicone elastomer of patient skin tone. This requires the presence of the patient to reproduce their skin tone into the silicone elastomer. The process requires hand mixing the silicone and color pigments and include frequent color check in a face‐to‐face encounter with the patient. The resultant mixture is affected by the color perception of the prosthetist, surrounding lighting conditions, and silicone processing temperature. After packing the mold, the silicone is heat‐cured for several hours. Once set, the silicone prosthesis is removed from the mold, finished by removing excess margin flashes, and delivered to the patient. It might need the addition of the extrinsic colorants via manual hand painting to maximize the esthetic result (Figure 14.1).
Such conventional approach is time‐consuming, laborious, requires a high degree of technical and clinical skills, patience, and can be expensive. Also, it is discomforting to the patients especially the part of taking physical impression of their defects (Feng et al. 2010). A comparative study indicated that eight hours were needed to fabricate a nasal prosthesis conventionally, while five hours were needed for the digital workflow process (Nuseir et al. 2019). Additionally, maxillofacial prostheses require frequent replacement as they deteriorate in color, fit, and mechanical properties upon use and when exposed to human precipitation and environmental factors (Hatamleh et al. 2016).

Figure 14.1 Illustrative steps showing construction sequence of an implant‐retained auricular prosthesis.
14.2 Digital Maxillofacial Prosthetics Workflow
It aims to replace the traditional steps involved in maxillofacial prosthetics, namely impression‐taking and processing, wax‐sculpting and prosthesis prototyping, mold‐making, skin color reproduction, and silicone prosthesis manufacture using the digital equivalents of 3D scanners, computer‐aided design/computer‐aided manufacturing (CAD/CAM), digital color spectrometry, and rapid prototyping technology or 3D printing.
14.3 Defect Digital Acquisition and Virtual Reproduction
The digital workflow requires the acquisition of the defect details at the 3D level. This can be achieved using medical or surface scanners. Medical scanning includes magnetic resonance imaging, CT, and cone beam computed tomography (CBCT) (Marro et al. 2016). The defect data is usually provided as Digital Imaging and Communication in Medicine (DICOM) format, which is exported and processed via a medical software into virtual 3D representation of the defect. Radiation dose usually determines the accuracy and resolution of the resultant 3D defect visualization as low‐resolution images may lead to surface discrepancies in relation to the scanned object while high resolution means exposure to a higher radiation dose (Marro et al. 2016). However, for intraoral and complex defects, the use of a CBCT scan is mandatory especially excision of malignant lesion for postoperative assessment (Zhao and Rao 2017).
Surface scanners are variable and include laser scanners, structured light scanners, facial scanners, and intraoral scanners. They are the most‐used devices for defect data acquisition (Cristache et al. 2021). Laser scanner moves a line of laser relative to the defect or structures being scanned. A charged couple device captures the resultant distortion of the light pattern and the defects surface coordinates are then calculated via triangulation language. Whereas structured light scanners project a light pattern onto the target defect or object surface, the projected pattern that represents the image of the object is then captured via video or photo cameras (Figure 14.2).

Figure 14.2 An example of a light scanner employed in scanning a cast model of a left ear.
Several algorithms for the range measurement of the 3D structured light scanner can be utilized. Mostly, the technology behind the scanner will employ triangulation to calculate the distance of projected light point or line(s) on the object to the sensor. It is accurate in performing distance and angle measurements. Both scanners have proven to be accurate and have been used successfully in digital acquisition for the production of auricular, nasal, and orbital prostheses (Subburaj et al. 2007).
Photogrammetry works by reconstructing 2D images into 3D visualization of scanned surfaces. It showed to be effective in performing distance measurements, but the quality of their 3D model surfaces resolution is low when compared with same models produced with CBCT (Naveen et al. 2018). Furthermore, laser scanners are incapable of registering deep undercuts or concavities present within a defect area as medical scanners do. Patients also need to stand still while their defect is being scanned otherwise misfits can occur. Thus, both types of data acquisition are usually needed for the same defect and registered by defining corresponding points on both scans (Cristache et al. 2021). Some studies proposed using two surface scan systems: stereophotogrammetry and light scanning in digital impression‐taking of an orbital defect and were matched using a specialized software that followed best‐fit algorithm provided by a color‐coded deviation map (Liu et al. 2019). Examples of such amalgamations are present in Figures 14.3 and 14.4.

Figure 14.3 Shows a patient with right orbital defect where his photogrammetry scan has been amalgamated with his CT scan.

Figure 14.4 A three‐dimensional image of a patient’s face (in silver) amalgamated with her soft tissue computer tomographic reconstruction (in red) to get an enhanced soft tissue view (large image).
14.4 Digital Defect Visualization and Reconstruction Design
Two different categories of software are used in the digital workflow of maxillofacial prosthesis construction: software for visualization of patients’ defect by processing scan data and design software (computer‐aided design [CAD]) to digitally sculpt the desired part. Additionally, skin tones are digitally reproduced, which is then integrated within the digital defect to facilitate defect reconstruction.
14.5 Digital Scan Visualization
It aims to visualize the patient and their defect digitally by the virtual reconstruction of the patient’s DICOM images (slices) data into visual 3D representation of the target tissues using a specialized medical software. It works by defining the Hounsfield units (HU) of the target structures that correspond to their physical density that is proportional to the X‐ray beam absorption/attenuation. A gray scale image is produced based on the radiation/attenuation coefficient of CT scan DICOM images reconstruction (DenOtter and Schubert 2021). Hounsfield units range varies from –1000 to 3000 and differs for soft tissue, hard tissue, teeth and metal structures, and the denser the structure is, the higher the HU range (Figure 14.5). The software allows automatic segmentation of structures that can be altered upon close inspection of targeted area (Abdullah et al. 2019). The software‐editing tools enable performing 3D operations of mirror imaging, uniting, merging, and subtracting 3D structures. The final 3D object is exported as a standard triangle language (STL) file to design the restorative prostheses. Such software can be commercially available or open source such as Mimics (Materialise, Belgium) and 3D slicer (The Slicer Community) being the most commonly used software respectively (Cristache et al. 2021).
The software enables importing surface scan to enhance visibility and planning of case. For example, Figure 14.6 shows importing a 3D image of a patient into his CT scan to simulate soft tissue reconstruction.
A review study reported scoping 64 digital literature sources related to maxillofacial prosthodontics digital workflow and referred to 20 different medical image editing software packages that were used in craniofacial reconstruction. The most commonly used proprietary software comprised the following (Cristache et al. 2021):
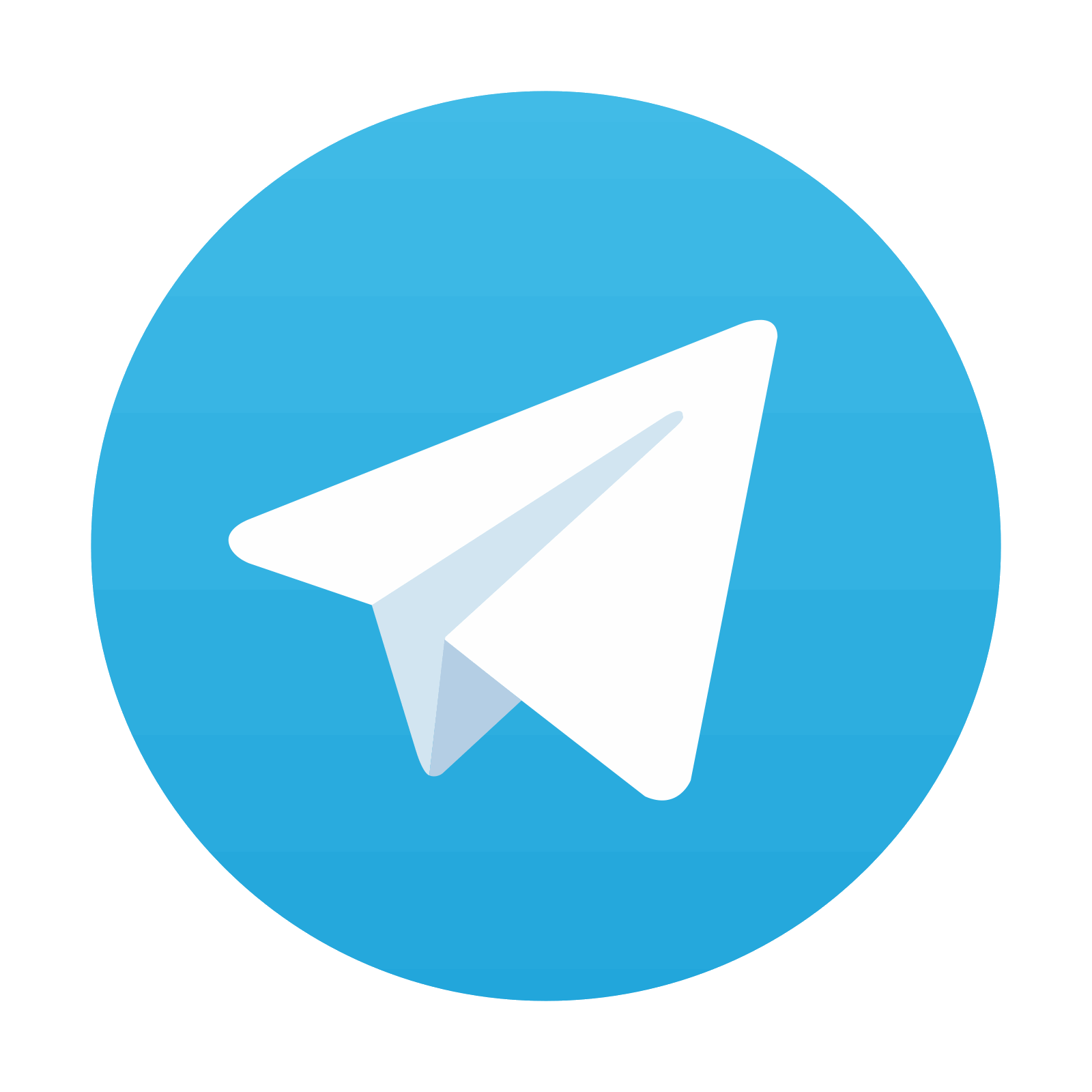
Stay updated, free dental videos. Join our Telegram channel

VIDEdental - Online dental courses
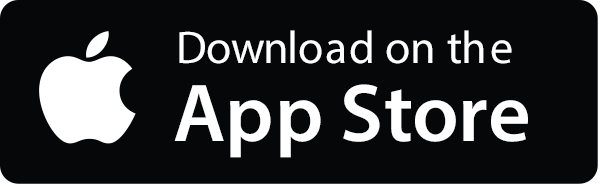
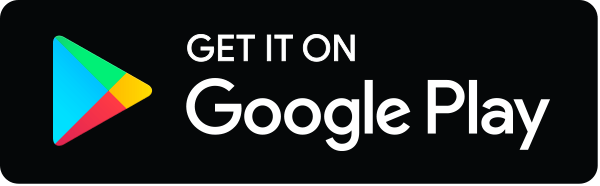