Stage
Reaction event
Response
1
Sodium and calcium hydrogen ion exchange on glass surface
Chemical
2
Dissolution of surface silica and formation of surface silanol
3
Condensation and repolymerisation of silanol to form SiO2-rich surface layer
Occurs in vitro or in vivo
4
Precipitation of amorphous calcium phosphate on the silica gel surface layer
5
Nucleation and crystallisation of amorphous calcium phosphate to carbonated apatite
6
Protein adsorption (growth factors, etc.) to the surface carbonated apatite layer
Biological
7
Action of macrophages to remove debris from surgical site
8
Attachment of mesenchymal stem cells on bioactive surface
9
Differentiation of stem cells to form bone-growing cells, osteoblasts
Occurs only in vivo
10
Generation of extracellular matrix by osteoblasts to form bone
11
Mineralisation of extracellular matrix to enclose bone cells, osteocytes
It has been shown that fibroblasts do not spread and proliferate on bioactive glass surfaces, contrary to what occurs on the surface of bioinert materials [139]. The exact mechanism is not clear, but may be due to the selective adsorption of serum proteins on the surface of bioactive materials. Bioactive glass containing a calcium phosphate-rich layer was found to preferentially adsorb fibronectin, which contains the integrin-binding arginine-glycine-aspartic acid (RGD) amino acid sequence for enhanced osteoblast adhesion [59]. Other researchers observed that the configuration of fibronectin adsorbed was different depending on the type of surface exposed by the biomaterial. A specific fibronectin conformation present on bioactive glasses that have reacted with simulated body fluid to form a surface Ca/P amorphous layer was found to induce very strong osteoblast adhesion [46]. This is important, since mesenchymal stem cells take time to migrate from their niches to a surgical site and arrive later than fibroblasts after a bioactive material is implanted. Thus, if fibroblasts proliferate, a non-adherent fibrous capsule forms, which inhibits interfacial bonding between the implanted material and the host tissue. When fibroblasts remain ‘quiescent’ along the surface of bioactive glass, new bone tissue can be produced upon differentiation of the mesenchymal stem cells and endothelial progenitor cells into osteoblasts and endothelial cells of the blood vessel wall, respectively.
A Bioactivity Index (IB) was introduced to rank the level of bioactivity of a specific material [73]. This index is defined as the time taken for more than 50 % of the interface to bond to bone (t 0.5) and is represented by IB = 100/t 0.5bb. Materials exhibiting an IB value greater than eight (e.g. 45S5 Bioglass®) will bond to both soft and hard tissues. Materials with an IB value less than 8 but greater than 0 (e.g. synthetic hydroxyapatite) will bond only to hard tissue [74]. The presence of silica was found to be crucial for a material to exhibit bioactivity. This is due to the partial dissolution of the material in an alkaline environment, which releases Si4+ ions to form the silica gel surface layer in stages 2 and 3 and creates silanol groups to act as sites for the nucleation of carbonated apatite from the amorphous calcium phosphate deposited over the silica gel layer in stage 5 (Table 4.1). Nevertheless, incorporation of increasing concentrations of silica in the glass resulted in a diminished rate of bioactivity. Increasing the silica content of the glasses decreases their dissolution rates by reducing the release of modifier ions such as Ca2+ and HPO4 − ions, which inhibits the development of the silica gel layer on the glass surface. Complete loss of bone-bonding ability occurred with further increases in SiO2 concentration, the exact concentration of which was dependent upon whether the glasses were melt-derived (>60 mol%) [76] or sol–gel reaction-derived (>90 mol%) [96]. The presence of P2O5 and Na2O was initially thought to be essential for glass-ceramics to be bioactive. However, sol–gel-derived CaO–SiO2 binary calcium silicate gel glasses were also found to bond firmly to bone tissues after intraosseous implantation [135, 136]. The information suggests that the absence of phosphate from most hydraulic calcium silicate cements does not preclude these cements from exhibiting bioactive behaviour. Conversely, partial substitution of CaO with Al2O3 in the binary CaO–SiO2 glass composition to produce ternary CaO–Al2O3–SiO2 glasses resulted in an Al2O3 concentration-dependent reduction/inhibition of the ability to form a calcium phosphate surface layer after exposure of these ternary glass formulations to simulated body fluid [16]. Addition of as little as three mass% Al2O3 completely inhibited the bone-bonding ability of bioactive glass [67]. The inhibitory effect of Al3+ on bone bonding was attributed to an increased resistance of the bioactive glass to ion-exchange surface reactions, to the precipitation of the multivalent ions as oxides, hydroxides or carbonates and to the shift of isoelectric point of the surface from negative to positive at physiological pH [173]. As tricalcium aluminate is a component of most hydraulic calcium silicate cements, the potential effect of aluminium on the bioactivity of these cements will be discussed in subsequent sections.
A functional bioactivity classification has been used to rate biomaterials designed for use as orthopaedic implants [164]. Class A materials demonstrate osteoproduction, which means that they can actively recruit cellular elements involved in bone formation (mesenchymal stem cells and endothelial progenitor cells) after implantation in a surgical site. These materials have IB values of 12. Class B materials show only osteoconduction [1]. These materials have IB values of 3–6 and function only as passive structural scaffolds for inward migration of cellular elements involved in bone formation. The term osteoproduction is similar to the contemporary terminology osteoinduction, meaning that primitive, undifferentiated and pluripotent cells are stimulated to differentiate into bone-forming and blood vessel-forming cell lineages [1]. Osteoproduction was used in the bioactive glass literature to describe bone proliferation resulting from the combined properties (i.e. osteoinduction, osteoconduction and/or osteogenic properties) of a grafting material. Based on this classification, a material is considered osteoproductive if it elicits both intracellular and extracellular responses at its interface (e.g. 45S5 Bioglass®) and osteoconductive if it triggers only an extracellular response (e.g. synthetic hydroxyapatite).
Release of inorganic ions by class A biomaterials may trigger intracellular responses [81]. Of the elements released by bioactive glasses, Si is known to be an essential element for metabolic processes associated with calcification of bone tissues [28] and induction of the apatite precipitation [38]. Dietary intake of Si has been shown to increase bone mineral density [84]. Orthosilicic acid stimulates type I collagen synthesis and osteoblast differentiation in human osteoblast-like cells in vitro [14, 127, 143]. Inorganic monomeric and polymeric silica/silicate have been shown to increase the expression of osteoprotegerin in osteogenic cells and modulate the crosstalk between osteoblasts and osteoclasts. Osteoprotegerin is a decoy receptor for the receptor activator of nuclear factor kappa B ligand (RANKL). Osteoprotegerin binding to RANKL on osteoblast/stromal cells blocks the RANKL–RANK ligand interaction between osteoblast/stromal cells and osteoclast precursors. This has the effect of inhibiting the differentiation of osteoclast precursors into mature osteoclasts. Thus, inorganic silica/silicate has the potential to stimulate osteogenesis in vivo by inhibiting osteoclast growth and differentiation [138]. Release of calcium ions favours osteoblast proliferation, differentiation and extracellular matrix mineralisation [98], activates Ca-sensing receptors in osteoblasts and increases expression of growth factors such as insulin-like growth factor-I (IGF-I) or IGF-II [104, 158]. Additional extracellular responses may be produced by adsorption of bone growth factor proteins, such as plasma-derived transforming growth factor-β1, by the surface silica gel layer. Bioactive glasses have also been shown to promote angiogenesis by stimulating the secretion of vascular endothelial growth factor by human fibroblasts [60, 61, 64, 95].
The information in this section represents only the tip of the iceberg of the humongous amount of research published on the bioactivity of glass and glass-ceramics. Nevertheless, the information provides the background for evaluating the bioactivity of hydraulic calcium silicate cements.
4.3 In Vitro Bioactivity of MTA
4.3.1 Direct Demonstration of In Vitro Bioactivity
Mineral trioxide aggregate (MTA) is the most well known and most thoroughly investigated of all the hydraulic calcium cements available to date. It is a clinker-derived Portland cement composed of different phases, including tricalcium silicate, dicalcium silicate, tricalcium aluminate, tetracalcium aluminoferrite and calcium sulphate. MTA is the first hydraulic calcium silicate cement patented for endodontic applications [155], which contains all the aforementioned mineral phases as well as bismuth oxide as a radiopacifier [24]. The aluminoferrite phase in the grey version of MTA is absent from the white version of MTA [124]. The compositions of other commercially available hydraulic calcium silicate cements designed for use as endodontic cements and sealers are covered in Chaps. 7 and 8.
Although bioactivity is not as esteemed property for industrial applications of Portland cements, in vitro bioactivity of white Portland cement has been reported after it was immersed in simulated body fluid for 7 days [36]. Using a combination of X-ray diffraction (XRD) and Fourier transform-infrared spectroscopy (FT-IR), the authors reported the formation of a layer of hydroxyapatite on the surface of white Portland cement after immersion of the set cement in simulated body fluid. Dissolution of portlandite (calcium hydroxide) and formation of calcite (calcium carbonate; reaction product of calcium hydroxide with atmospheric carbon dioxide) were also observed on contact of set white Portland cement with simulated body fluid. In another study, set white Portland cement was immersed in phosphate-containing fluid and the resultant calcium phosphate phase examined using XRD and FT-IR, in combination with scanning electron microscopy, transmission electron microscopy and electron diffraction [149]. The authors reported that the initial calcium phosphate phase formed was amorphous calcium phosphate. This precursor phase was subsequently transformed into calcium-deficient, poorly crystalline, B-type apatite (i.e. the PO4 3− groups in apatite being substituted by CO3 −). The authors concluded that the in vitro bioactivity of MTA materials is likely to be attributed to their Portland cement component. Identification of the amorphous calcium phosphate phase is of biological significance because the latter is the key intermediate calcium phosphate precursor phase that precedes biological apatite formation in in vivo osteogenesis [99–101]. As will be discussed below, identification of amorphous calcium phosphate precursors bridges the missing link in the sequence of stages that contribute to the in vitro bioactivity of hydraulic calcium silicate cements. In addition, by using the contemporary principles of biomineralisation, strategies have been developed with the use of polyanionic acid analogues of noncollagenous proteins for stabilising the amorphous calcium phosphate precursor phase to prevent its premature transformation into carbonated apatite. The resultant polyanionic acid-stabilised amorphous calcium phosphate precursors have been used experimentally for biomimetic intrafibrillar mineralisation of collagen fibrils in demineralised dentine [116]. Such a technology has potential applications in remineralisation of hybrid layers created by dentine adhesives to prevent their degradation by matrix metalloproteinases and cysteine capthepsins, as well as in the remineralisation of dentinal caries.
The two major components of Portland cements, tricalcium silicate and dicalcium silicate, have been produced separately using sol–gel reactions to create experimental, pure tricalcium silicate [160, 172] and dicalcium silicate [65] cements. These pure calcium silicate cements also demonstrated in vitro bioactivity by the precipitation of apatite crystallites on the cement surface after immersion in simulated body fluid. These results have led to the preparation of experiment bioactive tricalcium silicate [30, 48] and dicalcium silicate [25] cements for endodontic applications. The use of these experimental calcium silicate cements should alleviate potential concerns regarding aluminium-induced neurotoxicity [69, 141, 171] that may arise with the clinical use of clinker-derived tricalcium aluminate-containing hydraulic calcium (alumino)silicate cements.
By and large, the in vitro bioactivity observed in Portland cements and pure calcium silicate cements is recapitulated in MTA and the MTA-like clan of commercially available or experimental hydraulic calcium silicate cements designed for restorative and endodontic uses [5, 10, 15, 20, 25, 49, 51–58, 70–72, 128, 137, 142, 146, 147, 151]. These calcium phosphate reaction products were observed after the cements were immersed in simulated body fluid based on Kokubo’s formula or its modification [12, 89, 90], Dulbecco’s phosphate-buffered saline, Hank’s balanced salt solution or simply phosphate-containing fluid, with different degrees of reactivity. The ultimate reaction product formed on the surface of the set cements was, according to most of those publications, hydroxyapatite.
4.3.2 Mechanism of Action
The in vitro bioactivity of MTA and MTA-like materials is tissue independent and may proceed sequentially in stages that parallel those proposed for bioactive glasses. For comparative purposes, a similar sequence of events will be used in this discussion. These stages are schematically represented in Fig. 4.1.
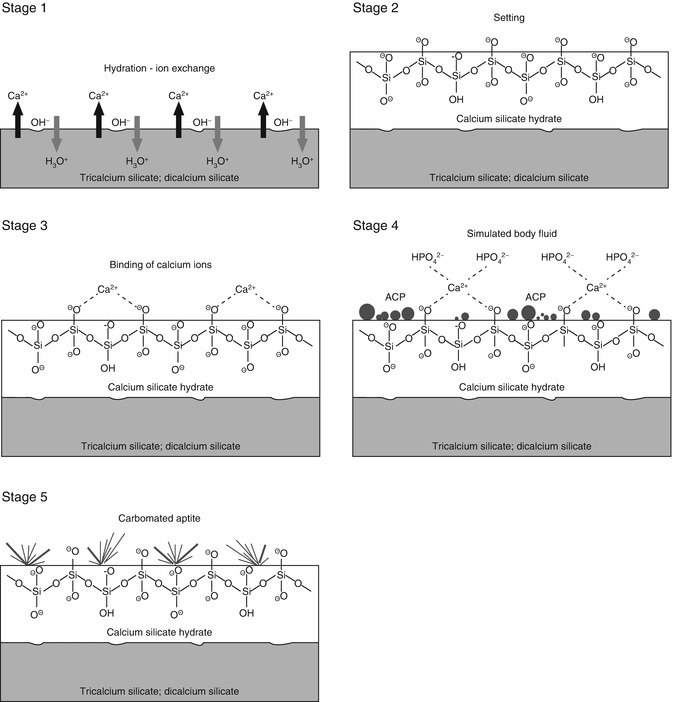
Fig. 4.1
A schematic of the five sequential stages of events that contribute to the manifestation of in vitro bioactivity of hydraulic calcium silicate cements after the latter is immersed in simulated body fluid (not to scale). ACP amorphous calcium phosphate
-
Stage 1 – Hydrolysis and ion exchange. Ion exchange occurs following hydration of the calcium silicate particles, with rapid exchange of Ca2+ with H+ or H3O+ ions from the aqueous mixing solution to form a solid–liquid interface [57]. Reaction of Ca2+ ions with OH− ions derived from water results in the formation of calcium hydroxide (portlandite) [22] that creates a highly alkaline environment. Although these reactions occur almost immediately after cement hydration, continuous release of Ca2+ and Si4− after initial setting, together with the release of minor amounts of Al3+, Fe3+ and SO42−, depending on the type of material, result in the formation of other inorganic mineral phases (Chap. 2).
-
Stage 2 – Formation of calcium silicate hydrate. Cation exchange increases the hydroxyl concentration of the solution. The surfaces of the calcium silicate particles are attacked by OH− ions in solution, resulting in hydrolysis of SiO4− group in an alkaline environment. The result is the formation of an amorphous calcium silicate hydrate phase on the surface of the mineral particles (Fig. 4.2a). Calcium silicate hydrate is a non-stoichiometric, porous, water-containing silicate gel layer [129] containing silanol (Si–OH) groups that forms the main binding phase in a set cement matrix.Fig. 4.2(a) Scanning electron microscopy (SEM) image of the surface of set white MTA powder showing formation of a calcium silicate hydrate layer (arrow) over the surface of the mineral particles after hydration of the powder. (b) SEM image of the surface of set white MTA powder after immersion in phosphate-containing fluid for 8 h. Amorphous calcium phosphate is deposited over the surface of the calcium silicate hydrate reaction phase in the form of spherule clusters (pointer). Some of the smaller amorphous calcium phosphate spherules are dispersed within the calcium silicate hydrate phase (open arrowhead)
-
This negatively charged surface attracts cations released into solution, such as Ca2+, via electrostatic interaction to decrease the total energy of the system, resulting in an increase of cations on the set cement surface:
(4.2)
-
This region, consisting of a charged surface and an equal but opposite charge in the solution, is called an electric double layer on which other substances may deposit under suitable conditions.
-
Stage 4 – Precipitation of amorphous calcium phosphate. When the set calcium silicate cement is immersed in a phosphate-containing solution, which contains hydrolysed HPO4 2− ions, electrostatic interaction occurs between the HPO42− ions with the Ca2+-rich CSH surface:
-
Continued release of calcium ions from the set cement into the phosphate-containing solution leads to supersaturation of Ca2+ and HPO42− ions in the solution, which in turn results in the formation of an amorphous calcium phosphate precursor phase in the solution. These initially formed subnanometre-sized amorphous calcium phosphate precursors are known as prenucleation clusters and have an average diameter of 0.87 ± 0.2 nm [41]. They remain relatively stable in the phosphate-containing solution in the absence of a nucleation-inducing surface. In the presence of a nucleation-inducing surface, aggregation of the prenucleation clusters leads to their densification near the cement surface, producing a ‘dense liquid’ CaP-rich phase [43]. Coalescence of the densified prenucleation clusters subsequently results in the deposition of globular amorphous calcium phosphate on the set cement surface (Fig. 4.2b), with the general formula Ca9(PO4)6-x(HPO4)x(OH)x [37]. Apart from the observation of amorphous calcium phosphate formation in Portland cement [149], amorphous calcium phosphate in the form of aggregated spherulites was also reported after ProRoot MTA (Dentsply Tulsa Dental Specialties, Tulsa, OK, USA), MTA Angelus (Angelus Soluções Odontológicas, Londrina, PR, Brazil), MTA Branco (Angelus) and MTA BIO (Angelus) were immersed in phosphate-buffered saline or Hank’s balanced salt solution [52, 128].
-
Stage 5 – Nucleation and transformation of amorphous calcium phosphate into carbonated apatite. In the presence of a nucleation-inducing CSH surface (≡SiO−…Ca2+… HPO4 2−), the amorphous calcium phosphate undergoes phase transformation over time into carbonated apatite [44, 117] (Fig. 4.3a, b). This transformation occurs via an octacalcium phosphate intermediate phase (Ca8H2(PO4)6 ·5H2O) [11, 45, 93, 156]. Whilst it is indisputable that apatite represents the endpoint of this phase transformation, the morphology of the calcium phosphate phases presented in the endodontic literature on in vitro bioactivity of hydraulic calcium silicate cements is highly variable, ranging from acicular, lathlike, petal-like, platelike, globular structures to needle-shaped [5, 10, 15, 20, 25, 49, 51–58, 70–72, 128, 137, 142, 146, 147, 151], with different Ca/P molar ratios (ranging from 1.33 to 1.67) (Fig. 4.4). Although it is possible that some of these represent calcium-deficient apatite with variable degrees of lattice substitutions by cations such as Na+ and Mg2+ and anions such as Cl−, it is likely some of these crystalline morphologies represent the intermediate octacalcium phosphate (OCP) phase described in classic calcium phosphate characterisation studies. Formation of an intermediate OCP phase by MTA or MTA-like materials was sparsely discussed in the endodontic literature except in a couple of studies [52, 128]. Indiscriminate use of the term ‘hydroxyapatite’ (HA) in most of these endodontic studies is reflected by the inadequate chemo-analytical techniques employed for characterising the calcium phosphate precipitates. The so-formed apatite is not stoichiometric HA but is carbonated apatite, which represents the biological apatite found in bone, cartilage enamel and dentine [32, 42]. Accurate characterisation of calcium phosphate phases requires the simultaneous use of XRD and FT-IR; the latter may be further complemented with micro-Raman spectroscopy [50, 92, 105]. It should be emphasised that CaP mineral phase information derived from XRD cannot be substituted by the peak designations derived from the combined use of FT-IR and micro-Raman spectroscopy. Even with the use of all these techniques, differentiation between octacalcium phosphate and apatite is extremely difficult [18, 19, 148] and often required high-resolution transmission electron microscopy of the crystalline lattice planes to determine the length of the unit cell axes and their crystallographic orientation [166]. The crystal structure of octacalcium phosphate has been described as an alternative stacking of apatite layers and hydrated layers along its [142] direction [18, 106]. The similarity of the apatite layer in OCP and the structure in HA provide geometrically favourable conditions for phase transformation from octacalcium phosphate to carbonated apatite. In a model of OCP–HA transformation, it has been suggested that transformation of OCP to HA occurs through epitaxial growth of HA on the OCP surface along the OCP[100]/HA[100] and OCP [001]/HA[100] crystalline planes [19]. Transformation of OCP to HA may be accomplished in solution through dissolution–precipitation or occur by solid-state transformation via one of the two processes [47]:Fig. 4.3(a) SEM image of the surface of hydrated white MTA powder after immersion in phosphate-containing fluid for 168 h. A crystalline calcium phosphate mineral phase (open arrow) is scattered around the calcium silicate hydrate-coated MTA mineral particles (energy dispersive X-ray analysis data not shown). Amorphous calcium phosphate spherules can no longer be observed. (b) High-magnification image of the calcium phosphate mineral phase in (a). These crystallites have short a-axis and b-axis but long c-axis, forming needle-shaped crystallites with hexagonal cross sections (open arrowhead)Fig. 4.4Characterisation of precipitates formed by MTA BIO after 2 months of immersion in phosphate-buffered saline. (a) SEM image showing the acicular nature of spherules (original magnification, 8,000×). (b) Energy dispersive X-ray (EDAX) spectrum for precipitates in (a) and semi-quantitative chemical composition showing their Ca/P molar ratio. (c) SEM image showing petal-like precipitates (original magnification, 1,000×). (d) EDAX spectrum for precipitates in (c) revealed a greater Ca/P molar ratio and lattice substitution of Na and Cl. (e) SEM image of compact lathlike precipitates (original magnification, 1,000×). (f) Semi-quantitative analysis of the EDAX data derived from (e) indicates that the precipitates have a Ca/P molar ratio of 1.61 with lattice substitution of Na, Cl and Mg. (g) X-ray diffraction pattern of the calcium phosphate precipitates obtained after 2 months of immersion in phosphate-buffered saline, revealing the presence of poorly crystalline apatite (Reprinted from Reyes-Carmona et al. [128], copyright 2009, with permission from Elsevier)
(4.4)
-
The first reaction represents solid-state transformation and may occur in experiments that examined desiccated calcium silicate cements after they are immersed in phosphate-containing fluids. The second reaction requires an additional supply of calcium ions and is more likely to occur in solutions containing calcium. Such a scenario may be found when there is continuous leaching of calcium ions from the set hydraulic calcium silicate cements after they are immersed in phosphate-containing fluids or serum.
Taken together, evidence is abundant in the literature showing that hydraulic calcium silicate cements exhibit in vitro activity. However, these studies were not performed as rigorously as those examining bioactive glasses. Although there have been attempts to compare the in vitro bioactivity of different commercially available or experimental hydraulic calcium silicate cements, most of the works were qualitative in nature. In particular, there was no adoption of a matrix such as the use of an in vitro version of the Bioactive Index, in systematically quantifying the percentage of the cement surface covered by calcium phosphate salts or the variation in thickness of the apatite or apatite-like mineral layer over time.
4.3.3 Indirect Evidence of In Vitro Bioactivity
Interestingly, in vitro bioactivity of hydraulic calcium phosphate cements was demonstrated in dentine instead of bone. Sarkar et al. [137] were the first to show the formation of an apatite-rich interfacial layer along the cement–dentine interface when grey MTA was placed on top of dentine and immersed in phosphate-containing fluid for 2 months in vitro. According to the authors, this interfacial layer was firmly attached to the dentinal walls with no observable gap between the interfacial layer and dentine. Subsequently, Reyes-Carmona et al. [128] reported the formation of a similar interfacial layer along the cement–dentine interface after ProRoot MTA, MTA Branco and MTA BIO were immersed in phosphate-buffered saline for 2 months in vitro. Apatite-rich tags were also demonstrated in the dentinal tubules and their lateral branches. Han and Okiji [70] demonstrated similar interfacial layers and taglike structures within dentinal tubules after white ProRoot MTA (Dentsply Tulsa Dental Specialties) and Biodentine (Septodont, Saint-Maur-des-Fossés, France) were placed in instrumented root canals of bovine incisors that were irrigated with ethylenediaminetetraacetic acid and sodium hypochlorite and followed by immersion in Ca- and Mg-free phosphate-buffered saline for up to 3 months.
The mechanism involved in formation of an interfacial cement–dentine layer with the use of hydraulic calcium silicate cements is different from what occurs in dentine bonding or with the use of glass ionomer cements. In the absence of adjunctive chemical treatment, it is not possible for an inorganic layer to bond chemically or even interlock mechanically with the surface of highly mineralised dentine, which consists predominantly of mineralised type I collagen. In dentine bonding, for example, bonding of adhesive resin monomers to dentine is achieved by demineralisation of the dentine surface with acid or acidic resin monomers to create a partially demineralised collagen matrix. Hydrophilic adhesive resin monomers infiltrate this collagen matrix and polymerise in situ to create a hybrid layer that bonds the pure resin layer above to the mineralised dentine below [122, 159]. In glass ionomer cements and resin-modified glass ionomer cements, chemical bonding occurs after treatment of the dentine with polyalkenoic acid. An interfacial zone or intermediate layer is created between the glass ionomer cement and dentine, which represents the summation of the infiltration of polyalkenoic acid and subsequent deposition of ion-exchange reaction products into the partially demineralised collagen matrix [150, 170].
In the case of calcium silicate cements, a new type of interfacial interaction known as the ‘mineral infiltration zone’ has recently been reported [6]. In this interaction, the highly caustic (alkaline) calcium silicate cement hydration products are responsible for the degradation of the collagenous component of intertubular dentine. A porous intertubular dentine layer is produced in which the collagen fibrils are depleted, leaving behind porous ‘mineral ghosts’ [102] that enable the permeation of high concentrations of calcium, hydroxyl and carbonate ions to form a hypermineralised layer [6]. Such a process has been termed ‘alkaline etching’ [6], to distinguish it from the ‘acid etching’ process associated with the use of dentine adhesives and glass ionomer cements. Prior to this report (Atmeh et al.) [6], a similar hypermineralised layer was observed on the of the resin-dentine interface (i.e. hybrid layer) when set white Portland cement was used to remineralise hybrid layers created in severely compromised bonded dentine [102]. Whilst this ‘mineral infiltration zone’ enables a calcium silicate cement to ‘bond’ to dentine, it is likely to be highly brittle because type I collagen, which is responsible for the toughness of biological mineralised tissues [8, 94], is destroyed by the alkalinity of the calcium silicate cements. It has been shown that hypermineralisation of cortical bone produces localised regions with reduction in fracture toughness that are prone to subcritical crack formation within the hypermineralised region [9]. These cracks provide sites for crack initiation following an impact. Similarly, dentine with high mineral/collagen ratios exhibits reduction in flexural strength and energy to fracture [132], which may contribute to increase in brittleness and decrease in fracture toughness of human dentine with age [83, 113]. To date, the long-term effect of taking advantage of the in vitro bioactivity of calcium silicate cements to bond to dentine is unknown, particularly for dental restorative procedures that involve placement of a fast-setting calcium silicate cement in dentinal cavities as a dentine replacement material [23].
4.4 In Vivo Bioactivity of Hydraulic Calcium Silicate Cements
As discussed in the introductory section, scientific evidence is lacking to support the assumption that a biomaterial that initiates the deposition of calcium phosphate salts on its surface after immersion in simulated body fluid will bond directly to bone following placement of the material in a surgical site [12]. Thus, it is appropriate to review the in vivo bioactive potential of hydraulic calcium silicate cements following their surgical implantation in appropriate animal models. Understandably, hydraulic calcium silicate cements employed in dentistry are not designed for complete filling of a surgical wound. However, it is important to see if the in vitro bioactivity reported for Portland cement and other hydraulic calcium silicate cements marketed for clinical use can be reproduced in vivo. Specifically, it is pertinent to identify if these materials can bond directly to bone without encasement by a fibrous connective tissue, the latter being the property of a bioinert material.
Because biocompatibility is not the subject of discussion in this chapter, studies that involved subcutaneous implantation of set calcium silicate cements will be excluded from the discussion. Likewise, histological studies that involved testing of calcium silicate cements as a root-end filling material will be excluded, as the tissue response involves migration of fibroblasts from the adjacent periodontal ligament to produce a layer of cementum over the material. Thus, only quantitative histological studies that involved the placement of calcium silicate cements as intraosseous implants will be examined. Of those studies, four studies [34, 35, 62, 63] that involved placement of calcium silicate cement-loaded polyethylene tubes into extraction sockets in a rat model were also excluded, as those implants were perceived to fit rather loosely within the surgical site. The results from the six studies selected based on these inclusion and exclusion criteria are summarised in Table 4.2.
Table 4.2
Studies on in vivo bioactivity (bone-bonding responses) of hydraulic calcium silicate cements following intraosseous implantation
Reference
|
Material investigated
|
Animal model
|
Surgical site
|
Number of intraosseous implants
|
Time period (days)
|
Delivery method
|
Results
|
||
---|---|---|---|---|---|---|---|---|---|
New bone apposition in direct contact with material
|
New bone separated from material by fibrous connective tissue
|
Mixed (incomplete direct bone contact)
|
|||||||
Number (%)
|
Number (%)
|
Number (%)
|
|||||||
[154]
|
Grey MTA
|
Guinea pig
|
Mandible
|
5
|
60
|
Teflon cup
|
1 (20 %)
|
4 (80 %)
|
NA
|
[153]
|
Grey MTA
|
Guinea pig
|
Mandible
|
10
|
70
|
Teflon cup
|
1 (10 %)
|
9 (90 %)
|
0 (0 %)
|
Tibia
|
11
|
80
|
5 (45.45 %)
|
5 (45.45 %)
|
1 (9.1 %)
|
||||
[111]
|
Grey MTA
|
Rat
|
Parietal bone
|
20
|
15
|
Freshly mixed; direct placement
|
0 (0 %)a
|
20 (100 %)b
|
0 (0 %)c
|
18
|
30
|
0 (0 %)a
|
14 (77.8 %)b
|
4 (22.2 %)c
|
|||||
18
|
60
|
2 (11.2 %)a
|
8 (44.4 %)b
|
8 (44.4 %)c
|
|||||
[133]
|
Grey MTA
|
Guinea pig
|
Mandible
|
13
|
14
|
Teflon cup
|
8 (81.5 %)
|
5 (38.5 %)
|
NA
|
13
|
84
|
7 (53.8 %)
|
6 (46.2 %)
|
||||||
Portland cement
|
13
|
14
|
7 (53.8 %)
|
6 (46.2 %)
|
|||||
13
|
84
|
10 (76.9 %)
|
3 (23.1 %)
|
||||||
[144]
|
Grey MTA
|
Guinea pig
|
Mandible
|
10
|
28
|
Teflon cup
|
Unspecified specimen numbers – fibrous capsule (absent/slight), new bone in close contact with material (extensive) for both time periods. Negative control (empty Teflon cup) – thin fibrous capsule for both time periods
|
||
12
|
84
|
||||||||
[126]d
|
Grey MTA
|
Rat
|
Femur
|
15
|
7
|
Freshly mixed; direct placement
|
15 (100 %)b
|
||
15
|
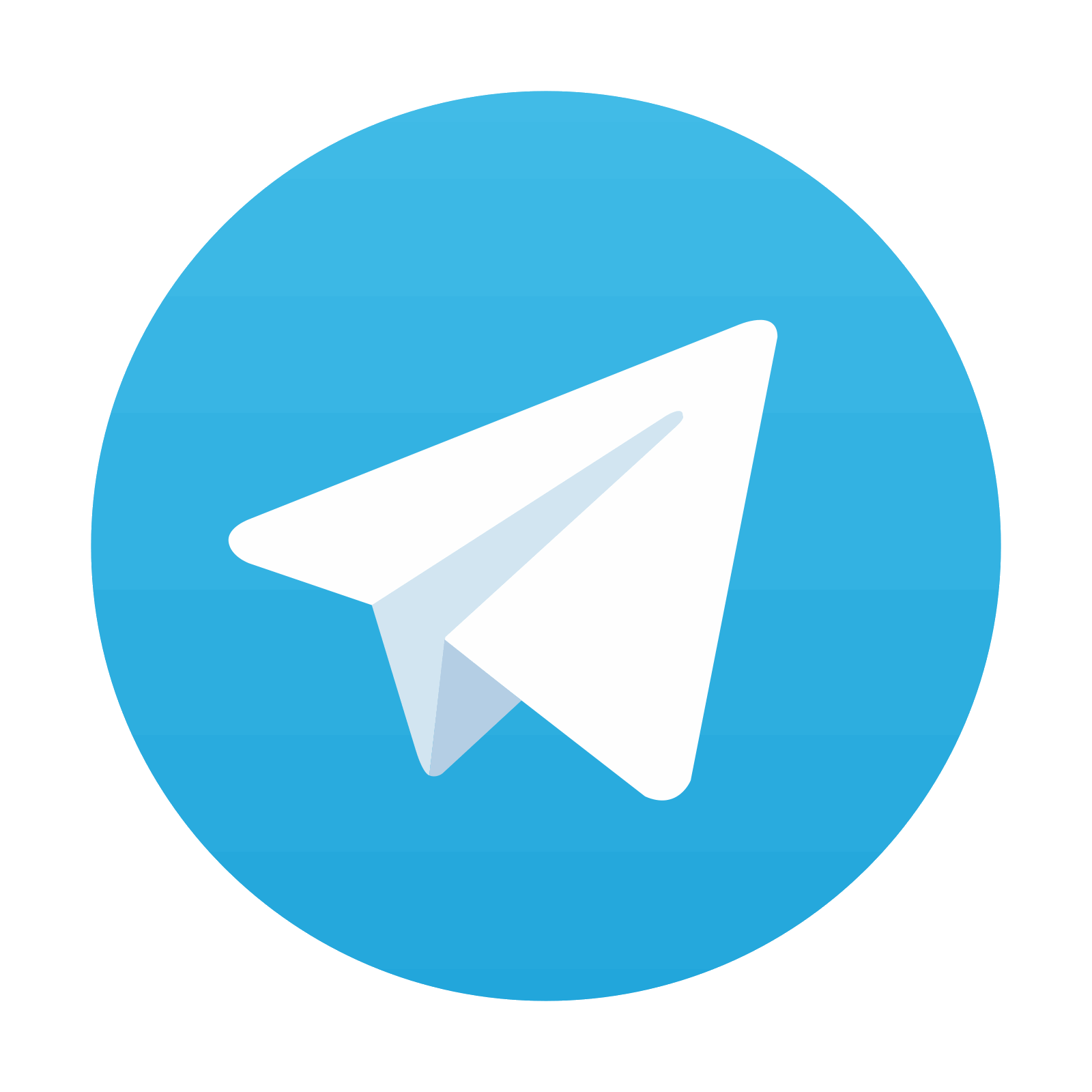
Stay updated, free dental videos. Join our Telegram channel

VIDEdental - Online dental courses
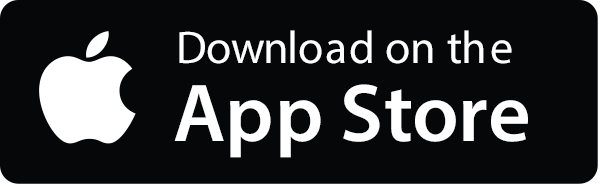
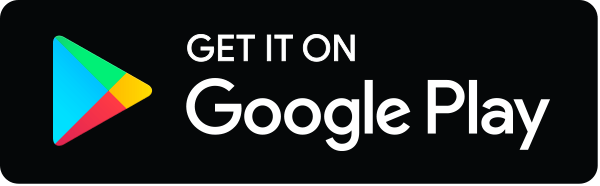