Highlights
- •
The composite filaments were successfully developed via melt-blending technique.
- •
AMP-PEEK filaments can serve as feedstock for 3D printing of patient-specific implants.
- •
The bioactivity of the composites significantly increased cell adhesion and proliferation.
- •
AMP-PEEK facilitate in vivo osseointegration and suitable bone regeneration capabilities.
Abstract
Objective
The aim of this study was to develop bioactive and osseointegrable polyetheretherketone (PEEK)-based composite filaments melt-blended with novel amorphous magnesium phosphate (AMP) particles for 3D printing of dental and orthopedic implants.
Materials and methods
A series of materials and biological analyses of AMP-PEEK were performed. Thermal stability, thermogravimetric and differential scanning calorimetry curves of as-synthesized AMP were measured. Complex viscosity, elastic modulus and viscous modulus were determined using a rotational rheometer. In vitro bioactivity was analyzed using SBF immersion method. SEM, EDS and XRD were used to study the apatite-forming ability of the AMP-PEEK filaments. Mouse pre-osteoblasts (MC3T3-E1) were cultured and analyzed for cell viability, proliferation and gene expression. For in vivo analyses, bare PEEK was used as the control and 15AMP-PEEK was chosen based on its in vitro cell-related results. After 4 or 12 weeks, animals were euthanized, and the femurs were collected for micro-computed tomography (μ-CT) and histology.
Results
The collected findings confirmed the homogeneous dispersion of AMP particles within the PEEK matrix with no phase degradation. Rheological studies demonstrated that AMP-PEEK composites are good candidates for 3D printing by exhibiting high zero-shear and low infinite-shear viscosities. In vitro results revealed enhanced bioactivity and superior pre-osteoblast cell function in the case of AMP-PEEK composites as compared to bare PEEK. In vivo analyses further corroborated the enhanced osseointegration capacity for AMP-PEEK implants.
Significance
Collectively, the present investigation demonstrated that AMP-PEEK composite filaments can serve as feedstock for 3D printing of orthopedic and dental implants due to enhanced bioactivity and osseointegration capacity.
1
Introduction
Orthopedic maladies, including but not limited to fractures, represent one of the most significant segments of global medical needs placing an economic burden on the healthcare system. In order to mitigate this issue, orthopedic biomaterials continue to be developed and refined based on patient-specific needs. As an example, polyetheretherketone (PEEK), a member of the polyaryl-ether-ketone family, has emerged as the leading high-performance thermoplastic candidate for replacing metallic implants . The present investigation represents the initial step in our long-term effort in developing patient-specific implants, made from PEEK composites. There are two motivating reasons. First, by tailoring the Young’s modulus of filled PEEK with that of cortical bone (18 GPa) PEEK-based implants can be made less prone to stress shielding, a well-known phenomenon that may ultimately lead to bone-implant debonding . Second, PEEK is biocompatible, causes neither mutagenic effects nor significant inflammation, and can be radiation sterilized. These are the reasons why, according to the literature, there is a growing interest in utilizing PEEK in oral implantology . Unfortunately, as compared to metallic implants, the major drawback of PEEK is its lack of osseointegration ability .
Extensive research has been done to enhance the bioactivity of polymeric biomaterials including PEEK . To that end, the development of various calcium phosphate (CaP) coatings have been reported . With the saturation of research on CaPs, there is a great deal of interest in alternate phosphates such as novel MgP compounds. A state-of-the-art review from our group explains the advantages of MgP Noteworthy, our group recently demonstrated the synthesis of multi-functional bioactive MgP coatings on PEEK . Broadly speaking, the reason for the reported favorable results is that magnesium ions (Mg 2+ ) are the fourth most abundant ion in mammals and participate in various cellular functions. For example, it is well-known that doping of Mg 2+ in CaPs enhances osteogenic proliferation and differentiation . However, the present work expands the research beyond crystalline MgPs to amorphous phases . It is known that amorphous calcium phosphate (ACP) has the tendency to crystallize . Incorporation of Mg 2+ ions in ACP inhibits crystallization . From that perspective, amorphous magnesium phosphate (AMP) is more stable in that phase and is gaining increased attention as a member of the range of MgP compounds.
Alternatively, attempts have been made to incorporate bioceramic particles within a polymeric matrix to enhance implant osseointegration . Several in vitro and in vivo studies of bioactive PEEK composites containing CaP particles were reported. Of note, micro- or nano-hydroxyapatite (HA) in the shape of spheroids or whisker , and β-tricalcium phosphate (β-TCP) have been used as bioactive second phases in PEEK. However, none of the previously reported studies used amorphous CaP or MgP, thus warranting further investigation. A processing-related disadvantage is that PEEK possesses higher melting point than conventional polymers. This is the reason why many PEEK composites have been fabricated by the powder route and not via polymer processing. As a result, composites needed further processing to achieve desired shapes, if at all possible. It is an acknowledged fact that powder consolidation technique is not so convenient from the perspective of 3D printing.
Here, to the best of our knowledge, we present the first comprehensive investigation aiming at the development of bioactive amorphous magnesium phosphate-polyetheretherketone (AMP-PEEK) composite filaments ( Fig. 1 ). These filaments will serve as feedstocks for three-dimensional (3D) printing in a subsequent effort. Our long-term goal is to fabricate bioactive, complex, defect-specific AMP-PEEK-based orthopedic and dental implants via 3D printing. Recently, we were successful in developing custom-designed PEEK based orthopedic implants via 3D printing and explored the pre-osteoblast behavior with them . However, before going ahead and printing AMP-PEEK structures, it is important to analyze the overall properties of the AMP-PEEK filaments by itself and determine the most suitable composition for 3D printing. Hence, the sole purpose of this study was to explore the development of AMP-PEEK composite filaments and evaluate their properties comprehensively. A key aspect was to determine whether AMP particles can be homogeneously distributed in PEEK matrix, and without any matrix degradation. In this context, composite filaments were formed out of PEEK and with varying AMP contents via melt-blending followed by a series of comprehensive physical, thermal, rheological, and biological evaluations. In detail, the in vitro cellular responses, including the metabolic activities of the proliferated mouse pre-osteoblasts, their differentiation ability, and expression of the osteogenic genes were determined. Moreover, histological and micro-computed tomography analyses were performed using a rat femur model of bone-implant osseointegration.

2
Materials and methods
2.1
Materials
AMP synthesis was carried out in-house, following the ethanol-assisted precipitation method previously employed by Babaie et al. . In brief, 11.52 g of magnesium nitrate hexahydrate (Mg(NO 3 ) 2 ·6H 2 O), 98% purity, Alfa Aesar, Tewksbury, MA, USA) was added to 100 ml of water and 100 ml of ethanol followed by proper stirring. This solution was then added rapidly, at 37 °C under constant stirring, to a solution containing 2.97 g of diammonium hydrogen phosphate ((NH 4 ) 2 HPO 4 , 99% purity, Fisher Scientific, Hampton, NH, USA) in 250 ml water, 45 ml ammonia (11 M) and 295 ml ethanol. The procedure resulted in the formation of precipitate which were collected, centrifuged and washed in ethanol. All the aforementioned steps were carried out within 15 min. Finally, precipitates were placed in an air-drying oven at 60 °C overnight to form fine powders. PEEK powder (particle size ca. 80 μm) was procured from GoodFellow (Goodfellow Cambridge Limited, Cambridge Science Park, Cambridge, England) and used without any further processing.
2.2
AMP-PEEK blending
Different compositions of as-synthesized AMP and PEEK powders were first mixed using a 100 W overhead stirrer (Mophorn, Huaian City, Jiangsu Province, China) for 3–6 min at room temperature. Table 1 contains the specimens’ code and their respective compositions. To obtain well-mixed AMP-PEEK composites, melt-blending was carried out using the twin-screw extrusion method. Briefly, blended powders of appropriate AMP-PEEK compositions were fed into a twin-screw extruder (Xplore MC5, Xplore Instrument BV, Geleen, Netherlands) in 3 g batches. The co-rotating screws had a length of 150 mm each, and a fixed rotational screw speed of 50 rpm was chosen. Residence time was varied depending on the AMP content ( Table 1 ). Four different extrusion temperatures, namely 360, 370, 380 and 400 °C were chosen to investigate the effect of processing temperature on the composites. The extruded filaments ( ϕ = 2 mm) were air-quenched and subsequently pelletized. For physicochemical and in vitro characterizations, specimen dimensions were 2 mm in diameter and 5 mm in length.
Specimen name | AMP (vol.%) | PEEK (vol. %) | Residence time (min) |
---|---|---|---|
0AMP-PEEK | 0 | 100 | 0 |
5AMP-PEEK | 5 | 95 | 6 |
10AMP-PEEK | 10 | 90 | 12 |
15AMP-PEEK | 15 | 85 | 15 |
2.3
Physico-chemical characterizations
To analyze the thermal stability of AMP, the as-synthesized precipitates were heat-treated at 400, 500, 600 and 800 °C in an air-furnace oven. The effect of heat treatment on AMP was determined using focused beam mono-chromated CuKα radiation (44 kV, 40 mA) X-ray diffraction (XRD, Ultima III, Rigaku, Tokyo, Japan) for phase analyses and scanning electron microscopy (SEM, S4800, Hitachi, Tokyo, Japan) for morphological characteristics. A gas displacement pycnometer (AccuPyc 1330, MicroMeritics, Norcross, GA, USA) was used to measure the density of the AMP-PEEK composites. Theoretical densities were calculated by the rule of mixtures. The phase compositions and functional group analyses were identified by XRD and Fourier transform infrared spectroscopy (FTIR, UMA-600 Microscope, Varian, Palo Alto, CA, USA), respectively. To analyze the level of AMP dispersion within the PEEK matrix, cross-sections of the extruded filaments were viewed under SEM. Elemental analyses were carried out with energy dispersive X-ray spectroscopy (EDS, INCA, Oxford) at 20 kV and 15-mm working distance. Three samples per group were tested for the aforementioned analyses.
2.4
Thermal properties
Thermogravimetric (TG) and differential scanning calorimetry (DSC) curves of as-synthesized AMP were measured using a simultaneous thermal analyzer (SDT Q600, TA Instruments, Dallas, TA, USA) with a heating rate of 10 °C/min. Melting temperatures and degree of crystallinity of the composites ( n = 3) were analyzed using DSC (PerkinElmer, Waltham, MA, USA). Approximately 5 mg of granulated PEEK or AMP-PEEK were exposed to the following step-by-step program in a pure N 2 environment, namely Hold at 40 °C for 3 min, Heat from 40 to 370 °C at 10 °C/min, Hold at 370 °C for 1 min, Cool from 370 to 40 °C at 100 °C/min, Hold for 2 min at 40 °C and Heat from 40 to 370 °C at 5 °C/min. Data were acquired specifically from the final step.
The degree of crystallinity by weight ( X CW (%)) was evaluated using the equation:
XCW(%)=HmWf×Hc×100
where H m is the melting enthalpy obtained from the DSC scan, W f is the weight fraction of PEEK in the composite and ▴ H c is the melting enthalpy of fully crystallized PEEK (130 J/g).
2.5
Rheological properties
Complex viscosity ( η *), elastic modulus ( G ′), and viscous modulus ( G ″) for PEEK and AMP-PEEK composites were determined using a rotational rheometer (ARES G2, TA Instruments, TA, USA). Oscillatory shear tests were conducted at 370 °C, under N 2 environment, using 25 mm parallel plate geometry with a 1.2 mm gap spacing between the plates. To find the range where the elastic and viscous modulus are independent of the applied strain ( i.e. , the linear viscoelastic range, LVR), dynamic strain sweep tests were performed in the strain range of 0.1–100% at a constant frequency of 10 rad/s (Fig. S1). For dynamic frequency sweep measurements, 10% strain amplitude (such that it was within the limits of linear viscoelasticity and the optimum torque range of the transducer) and 0.1–500 rad/s frequency range was selected.
2.6
Evaluation of in vitro bioactivity (simulated body fluid, SBF)
We used a modified version (t-SBF) of the original Kokubo’s composition (c-SBF) . Bare PEEK (control) or AMP-PEEK specimens ( n = 3/group) were placed in containers filled with 15 ml of t-SBF and kept in a thermostatic water bath at 37 °C for 7 days. The solution (t-SBF) was replenished every 24 h. Finally, after 7 days, the samples were retrieved, washed and dried at room temperature prior to further analyses. SEM, EDS and XRD (using the same operational parameters as described in Section 2.3 ) were used to study the apatite-forming ability of the AMP-PEEK composites.
2.7
In vitro cytocompatibility studies
Mouse pre-osteoblasts (MC3T3-E1, CRL-2593™, ATCC, Manassas, VA, USA) were cultured in minimum essential medium (MEM-α, Thermo Scientific), supplemented with 10% fetal bovine serum (FBS, HyClone) and 1% penicillin/streptomycin (0.2 g/ml) at 5% CO 2 and 37 °C. Triplicate samples were used per group, for each time point, were analyzed for all in vitro cell-related analyses.
2.7.1
Cell viability and proliferation
Thiazolyl blue tetrazolium bromide (MTT, Sigma Aldrich, St. Louis, MI, USA) was used to determine cytocompatibility. Sterile specimens (filaments of length 5 mm and diameter 2 mm), cells (2.3 × 10 4 cells/well) were seeded on PEEK (control) or AMP-PEEK and cultured for 4, 7, or 14 days. At specified time periods, 500 μl MTT stock solution (12 mM) was added to the culture, followed by 4 h incubation at 5% CO 2 and 37 °C. Dimethyl sulfoxide (DMSO) was used to dissolve formazan and OD 570 readings were recorded using spectrophotometer (BioTek Instruments Inc., Winooski, VT, USA).
2.7.2
Osteogenic gene expression (qRT-PCR)
In this assay, MC3T3-E1 cells were seeded at 90% confluency in T75 flasks and further cultured on PEEK or AMP-PEEK specimens for 4 or 7 days. TRIzol reagent (Invitrogen) was used to extract the RNA from these cells and complementary DNA was produced by reverse transcription using M-MLV reverse transcriptase (Promega). qRT PCR was performed using SsoFast EvaGreen Supermix (Bio-Rad) by a 2-step amplification program (30 s at 95 °C and 60 s at 62 °C) on a thermal cycler (Eppendorf). The 2 −ΔΔCT method was used to perform the relative quantification of mRNA of the genes and presented as fold-change compared to control samples. Glyceraldehyde 3-phosphate dehydrogenase (GAPDH) was used as the housekeeping gene.
2.7.3
Pre-osteoblast cell-material interaction
PEEK or AMP-PEEK specimens (filaments of 5-mm length and 2-mm diameter) were seeded with 6.5 × 10 4 cells/well. After a 4- or 7-day culture, the specimens were retrieved, washed in PBS and immersed in 3% glutaraldehyde solution for 1 h at room temperature. Then, the specimens were incubated in ascending ethanol solutions (30–100%) followed by soaking in hexamethyldisilizane (HMDS). Subsequently, the specimens were left to dry overnight in a fume hood and sputter-coated with Au for SEM imaging .
2.8
In vivo implantation studies
2.8.1
Animal model of osseointegration
For in vivo analyses, PEEK or AMP-PEEK filaments (10-mm in length and 2-mm in diameter) were used. Bare PEEK was used as the control and 15AMP-PEEK (hereafter referred as AMP-PEEK) was chosen based on its in vitro cell-related results. Animal experiments were approved by the University of Michigan Institutional Animal Care and Use Committee (IACUC, protocol #PRO00008502). Twenty-four adult male rats (Fischer F344), 16-week-old (300–320 g) were obtained from Envigo RMS, Inc. (Oxford, MI, USA). Animals were kept in cages in groups of three, housed at 21 °C ambient temperature and maintained in a light/dark cycle of 12/12 h. Previous to the surgical procedures, general anesthesia induced with inhalation isoflurane (Piramal, Pennsylvania, USA) (4–5%) was performed. During the surgical procedures, the anesthesia was maintained with isoflurane (1–3%) using a calibrated vaporizer. The femur regions were shaved and scrubbed with chlorhexidine (2%). Incisions (2-cm) were made in the medial parapatellar regions with displacement and remoteness of the muscle complex. Drill diameters ranging from 2.0 to 2.5 mm were sequentially used under constant irrigation with sterile saline to perform the proximal epiphysis and to access the intramedullary canal. Autoclaved PEEK or AMP-PEEK composite specimens were press-fitted in the femur along the longitudinal axis through a medial parapatellar arthrotomy, filling the entire medullary canal ( n = 4/group/time point). Right femurs received PEEK implants and left femurs received AMP-PEEK implants. Post-surgery, animals were medicated with non-steroidal anti-inflammatory drug (5 mg kg −1 Carprofen® base, Pfizer) subcutaneously. After 4 or 12 weeks, animals were euthanized, and the femurs were collected.
2.8.2
Micro-computed tomography (μ-CT)
After euthanasia, muscle tissue and epiphyses were removed. Bone/implants were fixed with 4% paraformaldehyde and non-destructive analysis of the newly formed bone at the implant interface was performed (Scanco μCT 100 Medical AG, Brüttisellen, Zurich, Switzerland). Samples were scanned with pieces rotation in 360°, using 70 kV, 114 μA monochromatic X-rays and 25 μm voxel size. The exposure time was maintained to be 500 ms and 3 frames averaging was recorded. The Scanco Medical System software was used for image reconstruction. A region of interest (ROI) around the implants was defined. This was used to calculate “bone volume” (BV) and “bone implant contact” (BIC).
2.8.3
Histology
For histological analysis, non-demineralized samples were prepared. Bone-implant blocks were fixed in 10% formaldehyde followed by gradual dehydration using a series of ethanol solutions (70–100%). Specimens were processed using a tissue processor (Leica ASP300, Buffalo Grove, IL, USA) and subsequently placed into a series of methylmethacrylate and dibutylphthalate with progressively higher concentrations of benzoyl peroxide. Samples were manually embedded in partially polymerized methylmethacrylate (PMMA) and allowed to cure at room temperature. Blocks were then hardened at 37 °C overnight. Tissues were sliced (∼300 μm in thickness) through the center of the implant, glued to acrylic slides and allowed to set for 24 h prior to polishing procedures. Sections were then reduced to a final thickness of ∼30 μm by grinding/polishing under water irrigation. Subsequently, sections were stained with toluidine blue and analyzed under an optical microscope. Images were captured at 20× magnification, and digital image analysis software (Image J v.1.45; National Institutes of Health, Bethesda, MD, USA) was used to measure the bone-to-implant contact (BIC) (overview at 2× and 4× with high magnification at 20× and 40×) (Olympus BX51, Melville, NY, USA).
2.8.4
Histomorphometric and BSE-SEM bone-implant contact analyses
Eight non-superimposing fields, corresponding to the bone/implant interface (four fields on each side of the implant), were captured by photographing each histological section at 20× magnification. The Image J software was used to measure bone-implant contact (BIC). In detail, bone-implant contact was calculated by subtracting BIC regions along the implant perimeter from the total implant perimeter. Results are reported as mean percentages and standard deviation. Immediately after histomorphometry analysis, the implant/bone interfaces were also examined by SEM (BSE-SEM, MIRA3, Tescan, Brno, Czechia) . EDS was also performed (MIRA3, Tescan, Brno, Czechia).
2.9
Statistical analysis
For physical, chemical, and in vitro analyses, wherever applicable, results were represented as mean of 3 specimens ± standard deviation. One-way analysis of variance (ANOVA) with Tukey’s test was conducted using the Minitab software to determine the statistical difference between groups and p < 0.05 were considered significant. For in vivo analyses, rat was the unit analysis. Data obtained from in vivo experiments were evaluated by two-way ANOVA and post hoc Tukey’s test. Differences were considered statistically significant when p < 0.05. The program GraphPad Prism version 8.0 was used for the analyses.
3
Results
3.1
AMP physical and thermal properties
Fig. 2 (a)–(c) present the phase, morphology, elemental and thermal analyses of AMP. As shown in Fig. 2 (a), the wide and broad hump in the diffraction pattern of as-synthesized powders indicate the formation of an amorphous material. The amorphous nature of the bioceramic was retained up to 500 °C. Heat treating at 600 °C caused AMP to crystallize and form farringtonite (Mg 3 (PO 4 ) 2 , PDF: 98-001-4443). Additionally, the wide hump pertaining to the amorphous nature was present. Heat-treating AMP at 800 °C resulted in the formation of single-phasic and highly crystalline farringtonite, as indicated by well-defined and sharp diffraction patterns. SEM image reveal irregular-shaped structures with edgeless boundaries ( Fig. 2 (b)). Further, the SEM micrograph ( Fig. 2 (b)) also denotes the presence of spherical particles either individually or coalesced with sheets. EDS analyses confirmed the presence of O, Mg and P with a 1.63–1.7 Mg/P atomic ratio. Fig. 2 (c) shows the TG and DSC results of as-synthesized AMP. The overall weight loss was gradual in nature and recorded to be 22%, most of it (20%) being from room temperature to 600 °C. The primary weight loss can be associated with the removal of the absorbed water molecules in AMP during heating. DSC showed an exothermic peak at 702 °C indicating crystallization of farringtonite.
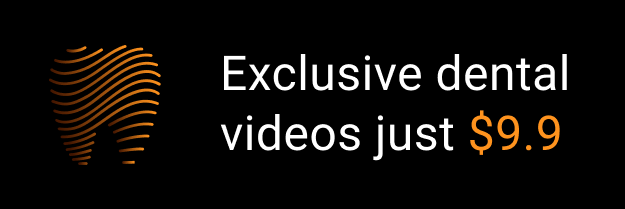