Chapter 7 The tooth-coloured restorative materials I
Resin composites
Learning Objectives
From this chapter, the reader will:
• Understand what resin composites are
• Understand the influence their constituents have on the material’s handling and its clinical applications
• Understand the properties of these materials and the significance of these on clinical manipulation and performance
• Understand their indications, contraindications and limitations
• Have an increased appreciation of how to use these materials to their best effect
• Know the names of currently available commercial products.
Introducing the Tooth-Coloured Restorative Materials
There is a range of directly placed tooth-coloured restorative materials available to the dentist. These materials form a continuum that links resin based composites to glass ionomer cements, with resin composite at one extreme and glass ionomer cement at the other and two hybrid materials in between (Figure 7.1).
The Resin Composites
Resin composites are widely used in dentistry and their applications are increasing as technology advances. As already mentioned in Chapter 6, dental amalgam is not necessarily the automatic material of choice for restoring posterior teeth in contemporary practice. The market is patient driven as most patients wish for the excellent aesthetics afforded by tooth-coloured restorations. Resin composite is the directly placed material of choice when the best aesthetic result is required. The early composite materials did not have long-term durability as they had a tendency to wear and showed staining. However, as materials and techniques have improved the longevity of resin composite restorations has increased. This chapter describes resin composites in detail and their applications and clinical manipulation.
The original tooth-coloured restorative materials (Table 7.1), the silicate cements and the acrylics, exhibited several shortcomings. The development of resin composite materials was aimed at overcoming these factors. As their properties have improved with time, they have tended to become ubiquitous materials, being used increasingly at the expense of other traditional materials.
Acrylics | Silicates |
---|---|
High water sorption | Low strength |
High coefficient of thermal expansion | Wash out |
Large polymerization shrinkage | Low pH |
Considerable microleakage | Pulpal irritation |
Extrinsic and intrinsic staining | |
Highly exothermic on curing | |
Wear | |
Poor colour stability |
Definition
A composite material is one that is composed of more than one different constituent. There are many composite materials in use in dentistry of which resin composites are one example. These materials are composed of a chemically active resin component and a filler, usually a glass or ceramic. The resin and the filler are bound together by a silane coupler. The structure of resin composite is illustrated in Figure 7.2, and the constituents of resin composite materials are shown in Table 7.2. The many component parts of a resin-based composite material are shown in Figure 7.3.
Table 7.2 Chemical constituents of resin composite materials
Ingredient | Examples | Reason for use |
---|---|---|
Filler (inorganic) | Glass | Provides strength |
Ceramic | Influences the optical properties of the material | |
Principal monomer | Bis-GMA | Forms polymer matrix |
Bis-EMA | Used as a primary monomer | |
UDMA | ||
Diluent monomer | TEGDMA | Reduces the viscosity of the main resin so that the material can be used clinically |
UDMA | ||
Silane coupling agent | γ-methacryloxypropyl-trimethoxysilane | Bonds the filler to the resin |
Photo-initiators | Camphorquinone | Initiator of polymerization reaction |
PPD | ||
Lucirin TPO | ||
Other chemicals for the curing process | Tertiary amine such as N,N-dimethyl-p-toluidene | Accelerator of polymerization reaction |
Ultraviolet stabilizers | 2-hydroxy-4-methoxybenzophenone | Prevents shade change over time due to oxidation |
Polymerization inhibitors | Monomethyl ether of hydroquinone | Prevents premature curing of the composite prior to use |
Radiopaque materials | Barium, strontium and lithium salts | Permits the material to be seen on radiographs (see Chapter 4) |
Pigments and opacifiers | Iron and titanium oxides | Varies the optical properties and the colour of the final material to achieve a good shade match |
UDMA, urethane dimethacrylate; TEGDMA, tri(ethylene glycol) dimethacrylate; PPD, phenylpropanedione; Lucerin TPO, ethyl 2,4,6 trimthylbenzoyldiphenyl-phosphine oxide.
Constituents of a Resin Composite
Resin component
Principal and diluent monomers
The main resin component is based on the chemical reaction of two resins, namely bisphenol A and glycidyl methacrylate, which form a chemical called bis-GMA (bisphenol A diglycidyl ether dimethacrylate), also known as Bowen’s resin after its inventor. It is a long chain monomer with a methacrylate group at either end of an aromatic spine. This chemical is highly viscous due to its high molecular weight and aromatic spine, which reduces mobility of the monomer so that it cannot be manipulated clinically (Figure 7.4).
Filler component
The resin, if used alone, shows a number of shortcomings:
• Reduced polymerization shrinkage
• Improved optical properties such as colour, fluorescence and translucency
• Radiopacity as heavy metals (such as barium) may be added as salts to the glass constituents before firing to produce the glass
• Less heat production during polymerization: the filler acting as a heat sink
Filler types
Resin composites have been classified by:
Glasses
A glass is an amorphous (non-crystalline) solid material. While there are many different chemical compositions of glass, the formulations used in resin composites are quite limited. Those which are used in these materials are listed in Table 7.3.
Once the glass has been produced by firing the various components of the glass together, the resulting solid mass is ground to the desired size of particle. Figure 7.5 shows a block of glass as supplied by the glass manufacturer and after it has been milled (ground).
Ceramics
• Zirconia-silica filler. This is manufactured in a sol-gel process. The particles have round edges and so more filler is able to be incorporated into the material. It is claimed to have very good translucency.
• Zirconium oxide. Groups of materials based on this oxide have the advantage that the composition can be finely controlled and may be varied by the manufacturer. Other benefits of this type of filler are limited at present and cost of production is considerably greater than the conventional filler production.
Sol-gel process: This is a process of glass or ceramic manufacture from the constituent oxides components and suitable volatile solvents. Once the volatile solvent has been removed the structure which is formed is known as a xerogel. On further heating, this structure consolidates into a form where it may be used as a filler. The resulting product is better in that it is more consistent in quality.
Macrofilled
The macrofilled composites were the first to be developed. The large size of the filler particles (range 15–35 μm maximum and minimum, 5–100 μm) meant that although the materials displayed good mechanical properties (high strength), they were notoriously difficult to finish to an acceptable level. This was because the particles would protrude above the surface in the resin and when the surface was polished these particles were displaced and a satisfactory polish was never achieved (Figure 7.6).
Microfilled
The semisolid mass is then heated to polymerize it and the result is a resin block with ‘seeds’ of colloidal silica embedded in it (Figure 7.7). This resin mass is then ground up to form ‘filler’ particles which are then mixed with unpolymerized resin to form the paste provided to the dentist. The inorganic phase, colloidal silica, in these materials does not exceed 45% by volume. However, the presence of already polymerized resin means that the polymerization shrinkage observed is not greatly above that for the other types of resin composite.
Hybrid
As the term suggests, a hybrid composite contains particles of various sizes and shapes. They were developed to try to gain all the benefits of the microfine and macrofilled resin composites. These products offer a higher filler density as the particles can get closer together and fit into each other so interlocking. This means that there is a decreased amount of resin. The structure of a hybrid resin composite can be compared with crazy paving (Figure 7.8 and Figure 7.9), with the stone paving slabs representing the particles and the cement grout the resin. It can be seen that they are remarkably similar although the hybrid has slightly more space between the particles. This is one of the limitations of the hybrid in that the resin viscosity tends to prevent the close apposition of the particles unlike the cement in the crazy paving which can be a much lower viscosity.
Under ideal conditions packing can be improved substantially with the resin acting as a binder. Figure 7.10 shows the ideal distribution of packing to achieve an optimum filler loading (a trimodal distribution). Here the large particles form the bulk of the restorative. In the spaces between them are smaller spheres of intermediate size. There still remain even smaller spaces between the larger and small spheres. If even smaller spheres are then used to fill those gaps, a trimodal distribution of particle sizes (large, medium and small) is established. The filler distribution in Figure 7.10 is 15 large (green), eight small (blue) and 32 smallest (red). These fillers form the bulk of the restorative. Manufacturers attempt to produce this type of distribution but are limited by the variable particle size within each category. It is also difficult to ensure that the distribution is uniform through the material.
As the particle size decreases, a further complication arises in that light is not reflected within the restorative and more shades and tinting agents are required to make the materials aesthetically pleasing. Modern resin composites are close to the maximum filler loading that is achievable, this being about 86% by weight (70–72% by volume). These heavily filled materials provide a reasonable surface and can be finished using fine diamonds burs, sanding discs and abrasive stones to produce a relatively smooth surface. The long-term performance is not ideal as the resin surface soon starts to wear away (Figure 7.11).
Nanofilled
The nanoparticles coalesce into nanocluster fillers, which are loosely bound agglomerates of these particles. Agglomerates act as a single unit, enabling high filler loading and high strength. These materials have the strength of a hybrid material but are easier to polish as the individual filler particles are much smaller. There are some materials which are conventional hybrid materials to which nanoparticles have been added to fill inter-particular space (see Figure 7.12).
Effect of filler loading
The aim of increasing filler loading is to make the mechanical properties of the resin composite closer to those of the filler. This means that the strength in compression goes up but that the material becomes more brittle. The resistance to wear is potentially increased but overfilling may lead to surface breakdown as there is inadequate resin to bind the filler together. The more filler that is added, the stiffer and more viscous is the paste, and manipulation can become much harder. Sometimes adaptation to the cavity margin and wall is compromised.
Silane coupler
When the filler is added to the resin, the resultant product actually becomes weaker than the original resin component alone, which clearly negates the objective of adding the filler. This is because no bonding occurs between the filler and the resin. Manufacturers therefore have to chemically coat the surface of the filler particles to facilitate their bonding with the chemically active resin. This chemical is called a silane coupler. The silane molecule facilitates the formation of a chemical bond between the resin and the filler. The principle is similar to silanation treatment, when ceramics are to be bonded to the tooth using a resin composite bonding system, for example in the bonding of ceramic restorations such as veneers (see Chapters 11 and 22). One example of a silane coupler in glass-filled resin composite is γ-methacryloxypropyltriethoxysilane (γ-MPTS), a vinyl silane compound. The silane molecule is bifunctional with groups that react with the inorganic filler and others that react with the organic resin, hydrophilic and hydrophobic groups, respectively. The γ-MPTS is acid activated and when reacted with the filler, the methoxy- groups hydrolyse to hydroxyl groups, which in turn react with hydroxyl groups on the filler. There is also a condensation reaction with the hydroxyl group of the hydrolysed silane. The silane coupler then bonds via its carbon double bond to the resin. The bond can be degraded by water absorbed by the material during clinical function.
Chemicals required for the curing process
• Chemically cured (sometimes referred to as self-cured)
• Dual cured, materials that are activated both by light and chemically.
Light cured resin composites
• A photo-initiator, usually a peroxidase or a diketone such as camphorquinone. More recently, novel photo-initiators have been considered such as phenylpropanedione (PPD) and Lucirin TPO (ethyl 2,4,6-trimethylbenzoylphenylphosphinate) (see Chapter 2).
• An accelerator, a tertiary amine such as that used in the chemically activated two-paste systems.
Mechanism of cure
On exposure to a sufficient amount of light energy at the correct wavelength of light, the monomers will polymerize to form a rigid cross-linked polymer, the various polymer chains linking via the methacrylate groups. The process of light curing is explained in detail in Chapter 2.
• An initial pre-gelation phase where there is considerable mobility of the polymer chains. This occurs during the first 8 seconds of a 10-second curing cycle. During the last 2 seconds of the curing cycle the material goes through the gel stage and become much stiffer. This is known as the post-gelation phase. About 85% of the conversion that will be achieved occurs in this period. After the material is considered to be clinically set some post-curing continues but this is very limited – not exceeding 15% – and the bulk of which is generally complete within 2 hours. Little change occurs after 24 hours.
It should be remembered that the polymerization reaction is anaerobic, that is, in the presence of air the material will only be partially cured. This explains why if no matrix is used to cover the surface of the restoration the surface will remain tacky and is then called the oxygen inhibition layer. This partly cured layer should be removed and the restorative trimmed back to the fully set material.
Radiopaque materials
The need for restorative materials to be radiopaque was discussed in Chapter 4. In many cases the filler particles contain heavy metal derivatives which are inherently radiopaque, such as barium, zinc, strontium and ytterbium. In those materials containing radiolucent filler, particles such as quartz or lithium aluminium silicate, and barium salts, can be added to convey radiopacity to the material. There is an ISO requirement (see ‘The regulation of dental materials and the International Standards Organization’ on the inside back cover of this book) that the radiopacity of these materials is equivalent to 2 mm of aluminium. However, there is variation in the radiodensity of materials above this which depends on the volume of radiopaque filler used.
Properties
Polymerization shrinkage
• A material which shrinks markedly will leave a gap at the tooth–restoration interface, thus allowing the passage of bacteria and oral fluids. This is termed microleakage (Figure 7.13).
• As is discussed in greater detail in Chapter 11, the bond achieved between the material and tooth is very good indeed. During curing, a large amount of shrinkage will cause high levels of stress to be built up in the remaining tooth structure, the tooth–restoration interface or the restorative material itself.
• It is also more difficult to manipulate the material to form good contact areas and stable occlusal relationships when the material will shrink (Figure 7.14).
The advantages of decreased shrinkage are summarized below:
• Reduced postoperative sensitivity
• Reduced stresses within the tooth tissue, at the tooth–restoration interface and within the material
• Reduced bulk or microfracture of tooth tissue
• Improved bonding between the resin, adhesive and the tooth due to greater dimensional stability.
Some manufacturers have changed the resin chemistry by changing the constituents of the resin. By attempting to eliminate the lower molecular weight monomers that exhibit greatest shrinkage on polymerization, the overall shrinkage of the resin can be reduced. Many products have as their resin component a mix of bis-GMA with bisphenol A polyethylene glycol diether dimethacrylate (bis-EMA) and UDMA, which shrink much less on curing that the original bis-GMA/lower molecular weight systems. By using this system, there is virtual elimination of TEGDMA and the higher polymerization shrinkage values seen with it. This resin is more hydrophobic and a slightly softer resin matrix is created, which may influence wear resistance. The resin base colour is lighter and the higher molecular weight leads to less shrinkage as there is greater distance between the methacrylate groups on the monomer chain.
Strategies to overcome polymerization shrinkage
Incremental build up allows for more effective and uniform polymerization and reduces total polymerization shrinkage (Figure 7.15), which may decrease stress generated at cavity walls and so reducing potential for debond gaps and cuspal deflection. It has, however, been shown that even with incremental build-up, once the final increment is placed the risk of cuspal flexure still exists. Proper placement of the first increment is the most important step and the dentist should ensure that it is fully polymerized as this increment is the furthest from the light.
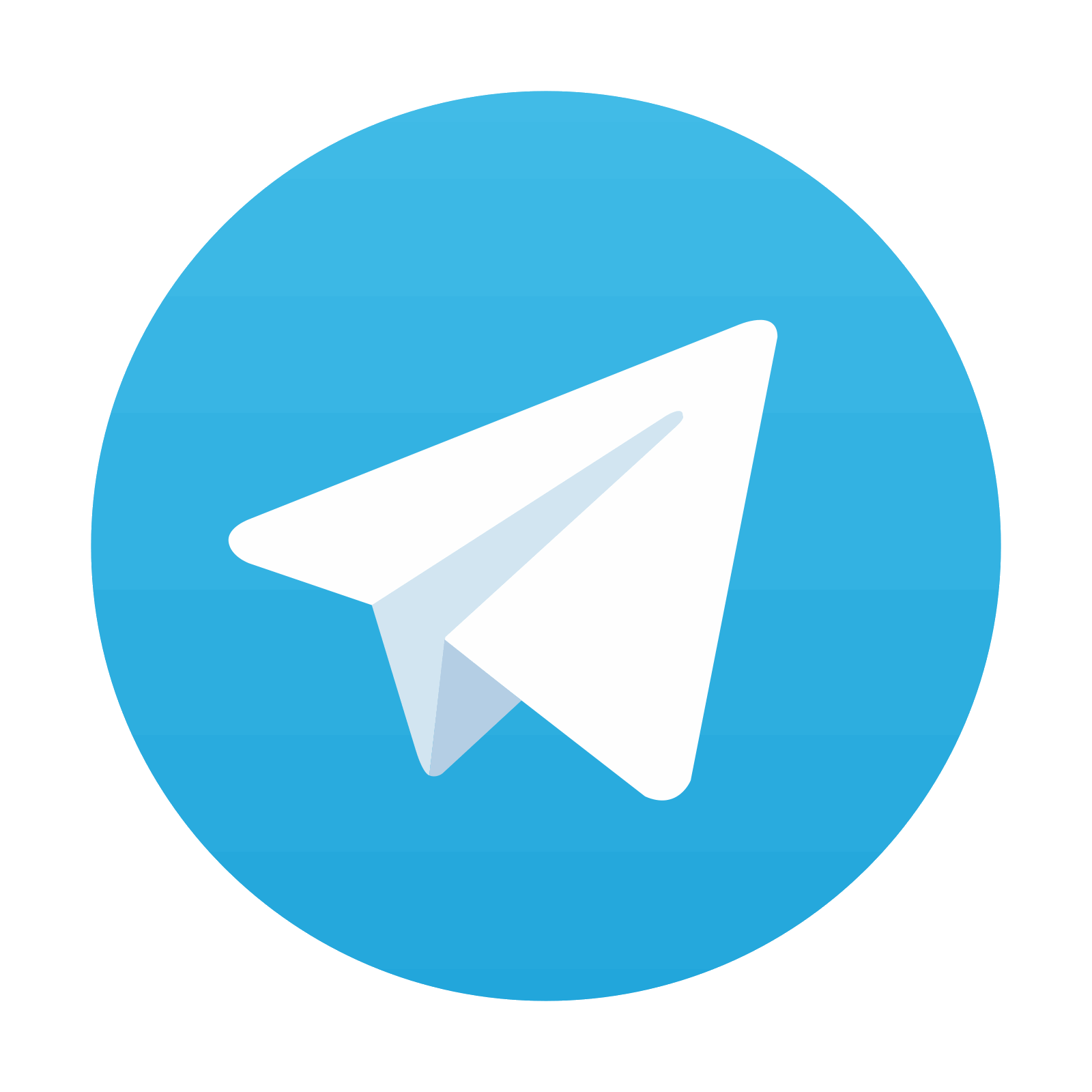
Stay updated, free dental videos. Join our Telegram channel

VIDEdental - Online dental courses
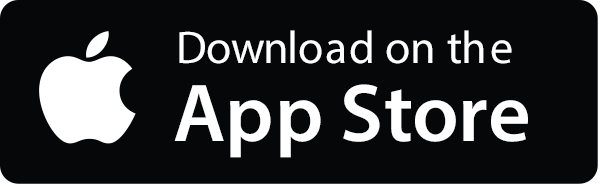
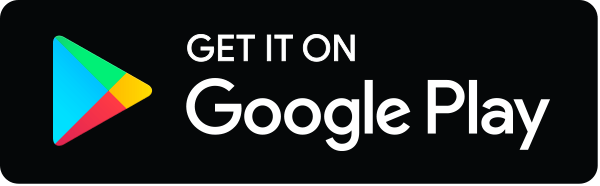