4 Orthodontic Wires
SEM photomicrograph of the surface of as-received Nitinol SE wire
Desirable Properties for Orthodontic Wires
Manufacturing of Orthodontic Wires
Clinical Selection of Orthodontic Wires
Introduction
Desirable Properties for Orthodontic Wires
Orthodontic wires, which generate the bio-mechanical forces communicated through brackets for tooth movement, are central to the practice of the profession. In the rational selection of wires for a particular treatment, the orthodontist should consider a variety of factors, including the amount of force delivery that is desired, the elastic (working) range or springback, formability or ease of manipulation, and the need for soldering or welding to assemble an appliance. In vivo corrosion, with release of metal ions, and the ensuing biocompatibility concerns for the patient are also important factors that will be discussed in Chapters 14 and 15.
Currently, orthodontists principally use wires of four major base metal alloy types: stainless steel, cobalt-chromium-nickel, nickel-titanium and β-titanium (Table 4.1). With the need to maintain a relatively large inventory, orthodontists must be concerned with the costs of the wires, which can vary considerably among wire alloys as well as among the companies that distribute these products. For example, selection of the more expensive nickel-titanium archwires can be somewhat offset by the reduction in the number of wire changes required during patient treatment.
Manufacturing of Orthodontic Wires
The starting point for the manufacturing of orthodontic wires is the casting of an ingot having the appropriate alloy composition. This ingot is then subjected to a series of mechanical reduction operations until the cross section is sufficiently small for wire drawing. The drawing for a round wire must be performed in a series of steps until the final desired diameter is achieved, since the alloy rapidly work-hardens during each step of this process. Generally, more than one company is involved in the complex wire manufacturing sequence.
Heat treatments are necessary during wire manufacturing to eliminate the extensive work hardening that occurs during the various stages of mechanical reduction. Information about these heat treatments is proprietary to the manufacturers, as are details about the reduction per pass, the number of passes, and the die and die lubrication materials. Special atmospheric conditions are needed for manufacturing the titanium-containing orthodontic wires because of the reactivity of these alloys with air. There is a tendency for the titanium-containing alloys to bind to the die or roller surfaces, resulting in increased surface roughness compared to other wire alloys. This can significantly affect the archwire-bracket friction, as will be discussed later.
Wire Alloys
Gold Alloy Wires
Historically, gold alloy wires were first used in orthodontic practice, although these noble metal wires have minimal use currently because of their much greater cost compared to the popular base metal wires. The gold alloy wire compositions were generally similar to those of the type IV gold casting alloys, and their modulus of elasticity was approximately 100 GPa. Thus, the gold alloy wires had elastic force delivery much less than that for stainless steel wires with the same cross-sectional dimensions and segment lengths (Table 4.1). Substantial hardening and strengthening of these gold alloy wires could be achieved by an appropriate heat treatment, which resulted in the same ordering phase transformation that can be used to provide hardening and strengthening for the types III and IV gold casting alloys.
Stainless Steel Wires
By the 1950s stainless steel alloys were used for most orthodontic wires. Stainless steel wires remain popular because of their favorable combination of low cost and excellent formability, along with good mechanical properties. These wires can be soldered and welded for the fabrication of complex appliances, although it is necessary to use solder to provide reinforcement to the weld joints.
The stainless steel alloys used for orthodontic wires are of the “18–8” austenitic type, containing approximately 18% chromium and 8% nickel (Table 4.1). While a report several decades ago showed that a 17–7 precipitation-hardenable stainless steel alloy had higher yield strength and greater resilience in bending than the commonly used stainless steel wire alloys, this alloy never achieved commercial popularity for orthodontic wires.
The chromium in the stainless steel forms a thin, adherent passivating oxide layer that provides corrosion resistance by blocking the diffusion of oxygen to the underlying bulk alloy. Approximately 12–13 wt% chromium is required to impart the necessary corrosion resistance to these alloys. The chromium, carbon, and nickel atoms (and atoms of other metals in the composition) are incorporated into the solid solution formed by the iron atoms. Since the nickel atoms are not strongly bonded to form some intermetallic compound, the likelihood of in vivo slow nickel ion release from the alloy surface is increased, which may have implications for the biocompatibility of these alloys.
Typically, stainless steel orthodontic wires are fabricated from AISI (American Iron and Steel Institute) types 302 and 304 alloys. Research using X-ray diffraction has shown that austenitic stainless steel orthodontic wires may not always possess the single-phase austenitic structure that is based upon a face-centered cubic (fcc) arrangement of the iron atoms. A two-phase structure was found for some as-received stainless steel wires (Fig. 4.1), where the austenitic (γ) phase was accompanied by a body-centered cubic (bcc) martensitic (α′) phase. Other as-received wires had the single-phase austenitic structure (Fig. 4.2). The X-ray diffraction patterns from these wires show the preferred crystallographic orientation that would be expected for wrought alloys. No peaks were evident from the (CrFe)4C phase, expected to be present in these alloys.
Fig. 4.1 X-ray diffraction pattern from 0.017 in × 0.025 in (0.43 mm × 0.64 mm) as-received TruChrome stainless steel wire (Rocky Mountain Orthodontics, Denver, CO, USA), showing a duplex structure of austenitic (λ) and martensitic (α′) phases. (Adapted from Khier et al, 1988)
Formation of the martensitic phase results in a substantial reduction in the modulus of elasticity, from about 200 GPa for fully annealed stainless steel alloys to about 150 GPa for heavily cold-worked alloys. Extensive cold working can increase the yield strength of these austenitic stainless steels from about 275 to over 1100 MPa. The foregoing range for modulus of elasticity in tension has been measured for stainless steel orthodontic wires of varying sizes, and indicates the variations in the levels of final cold-working mechanical deformation found in the as-manufactured wires. Heat treatment of some duplex-structure wires at three different temperatures of approximately 400 °C, 500 °C and 600 °C eliminated the α′ phase (Fig. 4.3), but other heat-treated wires maintained the two-phase structure found in as-received wires. There was little difference in the effects of the heat-treatment temperature on the X-ray diffraction patterns, and the wrought microstructure of the as-received wires was maintained after heat treatment at the highest temperature of 600 °C. The difference in the heat-treatment behavior was hypothesized to arise from variations in the carbon content of the wire alloys.
Heat treatment of stainless steel wires at temperatures between 400 °C and 500 °C is re-commended to eliminate some residual stresses resulting from wire manufacturing and to prevent premature breakage of complex appliances during assembly. Heat treatment of straight as-received wire segments yielded changes in the modulus of elasticity (E) in tension ranging from minimal to an increase of about 10% and increases in yield strength (YS) from less than 10% to more than 40%, depending upon the wire size. The modulus of resilience, which represents the total elastic biomechanical energy or spring energy in the wire, is given approximately by (YS) 2/2E. This expression can be used to estimate the changes in elastic spring energy resulting from the heat treatment.
Fig. 4.2 X-ray diffraction pattern from 0.050 inch (1.27 mm) diameter as-received Tru-Chrome stainless steel wire, showing a single-phase austenitic (γ) structure. (Adapted from Khier et al, 1988)
Fig. 4.3 X-ray diffraction pattern from 0.017 in × 0.025 in (0.43 mm × 0.64 mm) Tru-Chrome stainless steel wire after heat treatment at 480 °C, showing a single-phase austenitic (γ) structure. (Adapted from Khier et al, 1988)
Heat treatment of stainless steel orthodontic wires at temperatures above 650 °C must be avoided because rapid recrystallization of the wrought structure takes place, with deleterious effects on the wire properties. Heating stainless steel to temperatures between about 400 °C and 900 °C causes reaction of the chromium and carbon to form chromium carbide precipitates at the grain boundaries. Loss of chromium from the iron solid solution matrix of stainless steel depletes the chromium content near the grain boundaries to below the 12–13 wt% level required for passivation. This sensitization can cause the stainless steel alloy to become susceptible to intergranular corrosion.
The free-handed soldering of stainless steel orthodontic appliances should be performed rapidly with a well-controlled torch and the use of a flux. The solder joint is primarily formed by surface wetting and mechanical retention of the solder at the workpiece surfaces. In contrast, electrical resistance or spot welding of stainless steel causes melting and solidification of the alloy, with localized loss of the wrought microstructure and increased stresses in the surrounding heat-affected zone where joint failure is most likely to occur.
Cobalt-Chromium-Nickel Wires
A cobalt-chromium-nickel orthodontic wire alloy (Elgiloy) was developed during the 1950s by the Elgiloy Corporation (Elgin, IL, USA). This alloy, which was originally used for watch springs, is available in four tempers (levels of resilience) that are color-coded by the manufacturer: blue (soft), yellow (ductile), green (semi-resilient), and red (resilient). Discussions with the manufacturer indicate that all four alloy tempers have the same composition (Table 4.1); differences in mechanical properties arise from variations in the wire processing. As with the stainless steel alloys, the corrosion resistance of Elgiloy arises from a thin passivating chromium oxide layer on the wire surface.
The Elgiloy Blue alloy is very popular with many orthodontists because the as-received wire can easily be manipulated into desired shapes and then heat treated to achieve considerable increases in strength and resilience. This heat treatment can be performed easily with the aid of an electrical resistance welding apparatus, and the manufacturer provides a special paste that indicates when the appropriate conditions of temperature and time have been achieved. A furnace heat treatment for 10 minutes will achieve the same result.
It has been found that the maximum YS for straight, 0.41 mm diameter, wire segments is obtained with a heat-treatment temperature of about 500 8C. Heat treatment of four different loop configurations (open, helical open, closed, and helical closed) at this temperature decreased their mean deflection/force characteristics (i.e., increased the stiffness). This heat treatment causes complex precipitation processes that substantially increase the yield strength of the alloy.
Heat treatment of straight segments of Elgiloy Blue wires in several round and rectangular sizes yielded modest increases of about 10% in E and large increases of about 20–30% in YS. The other three tempers of Elgiloy have mechanical properties that are similar to tempers that are available with less expensive stainless steel wire alloys. These tempers, which are also responsive to heat treatment, are not as popular for orthodontics as the Elgiloy Blue wires.
Because of its “soft feel” (due to relatively low YS) during manipulation, orthodontists can mistakenly believe that as-received Elgiloy Blue wires have substantially lower elastic force delivery than stainless steel wires. In reality, the values of modulus of elasticity for the Elgiloy Blue and stainless steel orthodontic wires are very similar, as shown in Table 4.1. Other than the major differences in composition (Table 4.1), the stainless steel and Elgiloy Blue wire alloys have similar force delivery and joining characteristics. The Elgiloy Blue wires contain a comparable amount of nickel to that found in the stainless steel wires, which may present concerns about biocompatibility to some orthodontists.
Another interesting clinical use of Elgiloy Blue wires is fabrication of the fixed lingual quad-helix appliance, which produces slow maxillary expansion for the treatment of maxillary constriction or crossbite in the primary and mixed dentitions. The force delivery from an 8 mm activation for appliances fabricated from stainless steel and Elgiloy Blue wires of 0.032, 0.036, and 0.038 in (0.81, 0.91, and 0.97 mm) diameters was found to vary significantly with appliance size and wire diameter but was independent of alloy type. This result would be anticipated from the very similar values for the modulus of elasticity of the two alloys (Table 4.1).
Beta-Titanium Wires
A β-titanium wire for orthodontics is marketed by the Ormco Corporation (Glendora, CA, USA). The commercial name for this wire is TMA, which represents “titanium-molybdenum alloy“. The β-titanium alloy was conceived for orthodontic use about two decades ago by Burstone and Goldberg, who recognized its potential for delivering lower biomechanical forces compared to the stainless steel and cobalt-chromium-nickel alloys. Table 4.1 shows that the elastic modulus for the β-titanium wires is approximately 40% that of the stainless steel and Elgiloy Blue wires. Because of the much lower value of elastic modulus, despite lower values for yield strength, the β-titanium wires have significantly improved values of springback (YS/E), which markedly increase their working range for tooth movement.
A second clinical advantage of the β-titanium wires is excellent formability, which is due to their bcc structure. The addition of molybdenum to the alloy composition (Table 4.1) stabilizes the high-temperature bcc β-phase polymorphic form of titanium at room temperature, rather than the hexagonal close-packed α-phase. The X-ray diffraction pattern for a β-titanium (TMA) orthodontic wire in Figure 4.4 shows a single-phase bcc structure, with the broadened peaks and preferred crystallographic orientation expected for a heavily cold-worked alloy. The many slip systems available for dislocation movement in the bcc crystal structure account for the high ductility of the β-titanium wires. The zirconium and zinc in the alloy composition contribute increased strength and hardness, and their presence avoids the formation of an embrittling ω phase during wire processing at elevated temperatures. This wire processing can be problematic because of the reactivity of titanium, and there have been reports that some batches of TMA archwires are susceptible to fracture during clinical manipulation, despite the inherent excellent formability of the β-titanium alloy.
Fig. 4.4 X-ray diffraction pattern from 0.016 in × 0.022 in (0.41 mm × 0.56 mm) as-received β-titanium (TMA) wire. (An abstract describing this study is found in Brantley et al, J Dent Res, 1998)
The third clinical advantage of β-titanium is that it is the only orthodontic wire alloy possessing true weldability. As previously noted, welded joints for stainless steel and Elgiloy Blue appliances require additional mechanical reinforcement with solder. Detailed optimum settings for welding β-titanium wires with the capacitance (single-pulse) and transformer (multiple-pulse) welding apparatuses available to the orthodontist have been published.
The β-titanium wires are generally the most expensive of the orthodontic wire alloys, but the greater cost is frequently considered by orthodontists to be offset by the combined advantages of intermediate force delivery (Table 4.1) and the excellent formability and weldability when fabrication of more complex appliances is required. With increased interest in the biocompatibility of orthodontic materials, another important feature of the β-titanium wires is their absence of nickel that is present in the other three major wire alloy types. The excellent corrosion resistance and biocompatibility of β-titanium is due to the presence of a thin, adherent, passivating surface layer of titanium oxide (TiO2).
Scanning electron microscope (SEM) examination of β-titanium orthodontic wires has revealed relatively rough surfaces that are attributed to adherence or cold welding by the titanium to the dies or rollers during wire processing. This surface roughness contributes to the high values of archwire-bracket sliding friction measured in the laboratory, along with localized sites of cold welding or adherence by the wire to the bracket slots. A recently developed N+ ion implantation technique for β-titanium (Ormco) has markedly improved the measured values of in vitro sliding friction. The ion implantation yields high concentrations of nitrogen within a few tens of nanometers from the wire surface. Additional research is required to ascertain whether hard titanium nitride near-surface phases are formed during the ion implantation.
Nickel-Titanium Wires
The pioneer for the development of nickel-titanium wires for orthodontics was Andreasen, who published articles with colleagues advocating their use in the early 1970s. The first nickel-titanium orthodontic wire alloy (Nitinol) was marketed by the Unitek Corporation (now 3M Unitek, Monrovia, CA, USA). The generic name “nitinol” that is applicable to this group of nickel-titanium alloys originates from ni ckel, titanium and the Naval Ordnance Laboratory where the alloys were developed by Buehler and associates.
The Nitinol orthodontic wire offered a modulus of elasticity approximately 20% that of the stainless steel wires, along with a very wide elastic working range. This was particularly evident when this wire was tested in cantilever bending with 12.5 mm segments, where the permanent set did not exceed 58 after bending 908 by the ADA specification test procedure.
Two new superelastic nickel-titanium wires, Chinese NiTi (marketed as Ni-Ti by Ormco) and Japanese NiTi (marketed as Sentalloy by GAC International, Islandia, NY, USA) were subsequently introduced during the mid-1980s. When clinically realistic 6 mm segments (cor-responding to interbracket distances) of these wires were tested in cantilever bending, an extensive and nearly horizontal region (superelastic plateau) of constant bending moment was observed upon unloading (deactivation), as shown for the superelastic Ni-Ti alloy in Figure 4.5. (With the tension test, where there is uniform stress over the cross section, the loading and unloading superelastic plateaus are both horizontal.) These bending characteristics can be contrasted with those for the nonsuperelastic Titanal alloy (Lancer) in Figure 4.6.
Fig. 4.5 Cantilever bending curves for two sizes of as-received Ni-Ti superelastic wires. (Adapted from Khier et al, 1991)
Fig. 4.6 Cantilever bending curves for four sizes of as-received Titanal nonsuperelastic wires. (Adapted from Khier et al, 1991)
Fig. 4.7 Cantilever bending curves for 0.016 in (0.41 mm) diameter Ni-Ti wire after heat treatment at 500 °C and 600 °C for 10 minutes and 2 hours. (Adapted from Khier et al., 1991)
The springback (difference between the maximum deflection and the final deflection after deactivation) for as-received NiTi wires was found to be approximately 65° –70° for three superelastic alloys and 40° –60° for three nonsuperelastic alloys, respectively, with an original bending deflection of 80°.
Heat treatment of the Japanese NiTi wires at 500 °C was found to significantly alter the superelastic force plateau that occurred during unloading of three-point bending test specimens. This behavior has been exploited by GAC International, who offers three levels of force delivery by the Sentalloy wires. It was also observed that heat treatment at 600 °C eliminated the superelastic behavior. Subsequent experiments established that this heat treatment response at 500 °C and 600 °C occurred with other superelastic wire alloys (Fig. 4.7). The bending properties of nonsuper-elastic nickel-titanium wires are not affected by heat treatments over the same 500 °C to 600 °C temperature range.
In the early 1990s a NiTi orthodontic wire alloy (Neo Sentalloy) with true shape memory at the temperature of the oral environment was introduced by GAC International. Many manufacturers now offer similar shape-memory NiTi orthodontic wires, which might be considered to have an optimum combination of light force delivery and springback under clinical conditions. However, this hypothesis needs to be verified by clinical studies, and the original Nitinol wire remains popular. Some orthodontists have been concerned about biocompatibility of these wires because of their high nickel content.
Fig. 4.8 Nickel-titanium binary phase diagram, showing the region near the intermetallic compound NiTi that is relevant for orthodontic wires. (From Goldstein et al, 1987)
The complex metallurgical nature of the nickel-titanium orthodontic wires and its relevance to clinical applications has spawned many research investigations that are the principal focus of the remainder of this chapter. The nickel-titanium wires contain approximately equiatomic proportions of nickel and titanium, and are based upon the intermetallic compound NiTi (sometimes written as TiNi). Examination of the binary phase diagram (Fig. 4.8) reveals that some deviation from stoichiometry is possible for NiTi. X-ray energy-dispersive spectroscopic (EDS) analyses with the SEM suggest that commercial orthodontic wires are generally titanium-rich. The results of a recent study are shown in Table 4.2. Particles with dimensions typically less than 1–2 μm and containing a large atomic fraction of titanium were found in the surfaces and cross sections of all wire samples examined. The small size of these particles prevented accurate determination of their compositions by EDS.
There are two major NiTi phases in the nickel-titanium wires. Austenitic NiTi (here-after termed austenite) has an ordered bcc (CsCl-type) structure (sometimes termed B2), that occurs at high temperatures and low stresses. Martensitic NiTi (hereafter termed martensite) has been reported to have a distorted monoclinic, triclinic, or hexagonal structure, and forms at low temperatures and high stresses. The shape-memory effect (SME) is associated with a reversible martensite ↔ austenite transformation, which occurs rapidly by crystallographic twinning at the atomic level (Fig. 4.9). In some cases an intermediate R-phase having a rhombohedral crystal structure may form during this transformation process.
Wire Product | Surface Titanium Concentration (at%) | Bulk Titanium Concentration (at%) |
BioForce (GAC) | 50.5 ± 0.1 | 50.7 ± 0.4 |
Ni-Ti (Ormco) | 49.9 ± 0.1 | 50.2 ± 0.2 |
/> |
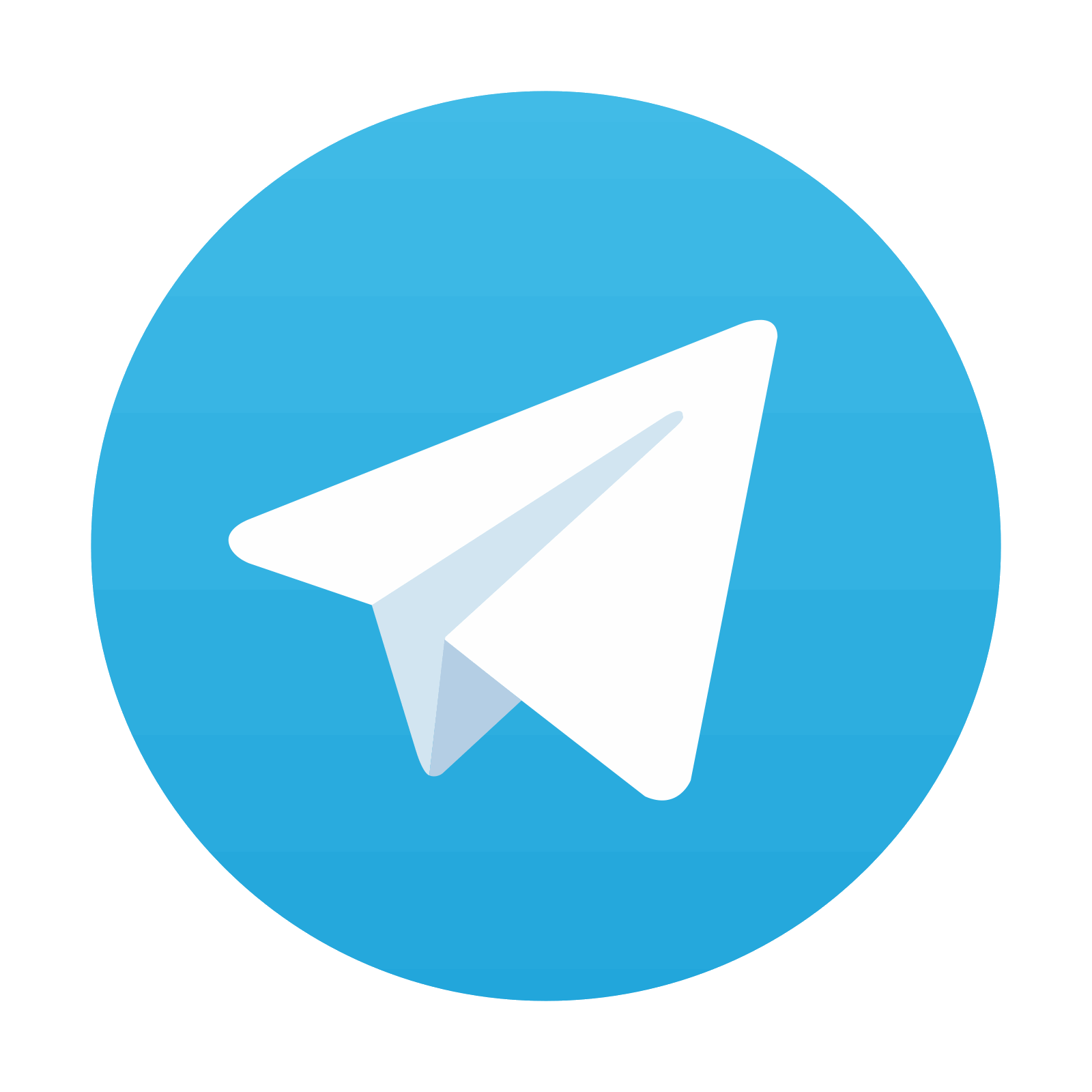
Stay updated, free dental videos. Join our Telegram channel

VIDEdental - Online dental courses
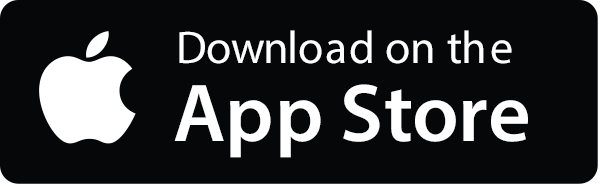
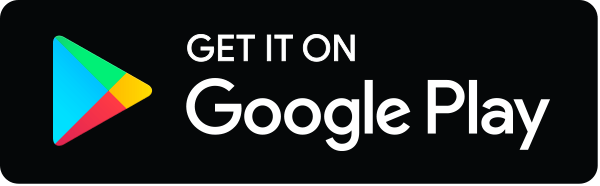