3
The central nervous system
Chapter contents
3.1 Introduction
The nervous system is an integrating system which acts rapidly by transmitting signals as electrical impulses over often considerable distances to coordinate bodily activities. The brain and spinal cord make up the central nervous system (CNS); incoming information travels in ascending (sensory) tracts that link the spinal cord to the brain and outgoing information passes down descending (motor) tracts linking the brain to the spinal cord. The CNS integrates responses to incoming information and sends the information to effector tissues (usually striated or smooth muscles or glands). Incoming and outgoing information is carried to and from the periphery to the CNS via 12 pairs of cranial nerves connected to the brain and 31 pairs of spinal nerves connected to the spinal cord; they constitute the peripheral nervous system (PNS).
Sensory (afferent) information from the external environment is obtained through the organs of special sense in the eyes, ears, nose and tongue, and skin and mucosa lining bodily cavities: we are aware of these stimuli. Information from internal sources is equally important and vital for maintaining homeostasis, but we are usually unconscious of it; for example, blood chemistry must be monitored as must the degree of stretch of internal organs. Proprioception, knowledge of the state of muscles and joint position, as described in Section 2.4.1, is another important source of internal information as are our cognitive processes. Motor (efferent) stimuli are conveyed from the CNS to effector tissues through the cranial and spinal nerves of the PNS.
The peripheral nervous system is further subdivided into the somatic and autonomic nervous systems. The somatic nervous system receives and transmits external sensory stimuli to the CNS and conveys motor responses to striated muscles. The autonomic nervous system conveys motor stimuli to smooth muscle and glands and is responsible for homeostasis. The autonomic nervous system has three subdivisions, the sympathetic, parasympathetic, and enteric systems; the first two act on many systems in an antagonistic manner where one system stimulates activity and the other suppresses it. The enteric system plays a role in the control of gut motility.
3.2 The cellular components of the nervous system
Neurons are the basic cellular units of the nervous system. As the principal function of the nervous system is conduction of electrical signals over considerable distances, neurons are highly specialized for this function. Neurons have:
• A specific shape with long cellular extensions;
• Highly specialized membranes to control ionic movements to allow electrical activity to spread along the cellular extensions;
• A very specialized internal transport system to distribute cellular metabolites along the processes.
The general shape of neurons is shown in Figure 3.1. Note first of all, the relatively large cell body near the top of the picture; this contains the nucleus and the intracellular organelles necessary for synthetic functions so is similar to any other cell. What make neurons special are the long processes that emanate from the cell body. Dendrites are short multiple processes that branch extensively from and transmit impulses towards the cell body. Compare the dendrites in Figure 3.1 with the other process, the axon, which transmits impulses away from the cell body. Axons are generally much thicker than dendrites and there is usually a single axon arising from the cell body; it may branch, but often at some distance from the cell body or as it nears its target. Axons may be extremely long. The cell bodies of neurons conveying information to muscles in the sole of your feet originate in the lower regions of the spinal cord in your lower back. Think of your inside leg measurement from when you last bought a pair of jeans, then add another 9 inches or about 20 cm; that is how long axons can be!
3.2.1 Nerve action potentials
We need to consider some cellular physiology to understand how neurons can transmit signals. All cells in the body possess ion channels in their membranes that allow ions to pass through so that the distribution of positively and negatively charged ions balances out between the inside of the cell and the surrounding extracellular fluid. The most common positively charged ions are sodium (Na+) and potassium (K+), and chloride (Cl−) is the most abundant negatively charged ion in bodily fluids. If positive and negative ions are equally distributed between the cell and the surrounding fluid, positive and negative charges balance out so there is no electrical voltage difference across the membrane. If neurons are going to be able to conduct electrical impulses, the distribution of ions must be uneven so that there is an electrical potential difference across the membrane (the membrane potential). This is a state of polarization. Figure 3.2A shows the distribution of ions in a neuron at rest. The resting membrane potential across neuronal cell membranes is about –70 mV; this is achieved by forcibly pumping Na+ ions out of the cell. Sodium channels are closed to prevent Na+ ions getting back into the cell. The removal and exclusion of Na+ ions leaves a surplus of Cl− ions inside making the inside of the cell negative with respect to the outside.
Fig. 3.1 A schematic diagram of a typical neuron.
Figure 3.2B shows what happens when a neuron is stimulated. Na+ ion channels open and allow the excess Na+ ions to rush into the cell, changing the potential across the membrane from negative to positive. If enough Na+ ions get in to change the potential difference between the inside and outside of the cell to around + 15 mV, the threshold potential is exceeded and the electrical activity will become self-generating along the dendrites and axons. This is called an action potential or depolarization (or ‘firing’ or nerve conduction). If the threshold potential is exceeded, the neuron will fire; if it is not, it will not generate an action potential. Neurons are basically on–off switches; they can only be on or off. This is known by physiologists as the ‘all or none’ rule of nerve conduction.
How does the movement of ions start and lead to an action potential? Ion channels in cell membranes can be opened or closed by three basic mechanisms. The most common ligand-gated channels are opened by a chemical signal (for example, a hormone or some other intercellular messenger) binding to a receptor on the cell membrane that opens the channel. Receptors are usually very specific for particular ligands. Mechanically gated channels are opened by mechanical changes such as pressure or tension; this is how a touch on your skin is converted into electrical activity in neurons. Finally, and most important in the context of generation of action potentials, ion channels may be opened by changes in voltage across the cell membrane; these are voltage-gated channels. The sequence of events is shown in Figure 3.2B. Thus a touch on your skin will cause mechanically gated sodium channels to open and cause depolarization of the neuron at a particular point. As the membrane potential changes in this area, it will cause voltage-gated channels in adjacent areas to open and the action will be propagated along the neuron by opening of further channels. We can now see the chain of events that generate an action potential, usually in dendrites or the cell body, in a single neuron which will propagate along the axon. We must now consider what happens when the action potential reaches the end of the axon.
3.2.2 Synapses
Axons terminate by forming specialized intercellular junctions with dendrites and/or the cell body of another neuron or with effector tissues such as muscles or glands. The junctions between neurons are called synapses and those between neurons and muscle are neuromuscular junctions. Electrical activity cannot simply jump from one neuron to another or from neuron to effector. This is absolutely vital in preventing the electrical activity spreading at random and generating total chaos in the nervous system. We have already seen that neurons will either generate an action potential or they will not—the ‘all or none’ rule. It follows that if a neuron is conducting an impulse, there is no physiological way to stop the neuron conducting. Along the chain of neurons that are required to get information from one part of the brain to another or from sensory receptor to effector, the only places where information may be modified are at synapses.
Figure 3.3 is a diagrammatic representation of a synapse. The first thing to notice is the synaptic cleft, a space between the axon of one neuron (the presynaptic neuron) and the dendrites and cell body of another (the post-synaptic neuron). This space is about 400 nm wide, minute, but sufficiently wide to prevent electrical connection. Observe in Figure 3.3 that the axons of the presynaptic neuron are expanded into synaptic boutons occupied by synaptic vesicles that contain neurotransmitters which are chemical messengers that allow communication between the two neurons. When an action potential reaches this zone of the neuron, the ion channels change to voltage-gated calcium channels. As the wave of depolarization opens these channels, calcium flows into the neuron and facilitates the fusion of the synaptic vesicles with the cell membrane, allowing release of the neurotransmitter into the synaptic cleft. Notice the structures drawn on the post-synaptic neuron in Figure 3.3. The neurotransmitter will cross the synaptic cleft and attach to membrane receptors on the post-synaptic neuron, opening ligand-gated ion channels as they do so. If the ion channels thus opened allow Na+ ions in, then a wave of depolarization will be set in train as described above. If, on the other hand, the channels were Cl− channels, then chloride would enter the post-synaptic neuron, making its membrane potential more negative and therefore, more difficult to depolarize (see Figure 3.2). Thus some neurotransmitters will be stimulatory whereas others will be inhibitory, depending on the type of ion channels they open. Any post-synaptic neuron has synaptic connections with several presynaptic neurons so the final balance between inhibitory and stimulatory signals received determines whether a neuron fires or not.
Fig. 3.2 Ionic changes across neuronal cell membranes. A) At rest; B) During depolarization; C) At the synaptic bouton.
Fig. 3.3 A schematic diagram of a neuronal synapse.
3.2.3 Nerve myelination
Neurons, as we have already seen, may have extremely long processes. They will become less efficient at conducting impulses over long distances unless they are insulated just like electrical cables on domestic appliances. Another advantage of insulation is that it minimizes the risk of cross-talk between neurons travelling side by side. The main insulating material used in the nervous system is a lipid called myelin that is wrapped around the axons. Some neurons are covered in this way and are therefore described as myelinated neurons whereas others do not have these layers and therefore are unmyelinated neurons. Myelin is not produced by neurons themselves, but by the non-excitable supporting cells of the nervous system called neuroglia or glial cells. Glial cells are, however, not simply insulating cells. They are also involved in the regulation of energy and metabolites reaching neurons. Neurons are very active cells and have large energy requirements. Something like 25% of the energy produced by your body is being used by your brain to process and control whatever your body is doing. The percentage may go even higher if you are reading this while sitting in a chair or lounging on your bed which requires little muscular effort.
Neuroglial cells outnumber neurons in the CNS by about ten to one. They comprise:
• Astrocytes;
• Oligodendrocytes;
• Microglia;
• Ependymal cells.
Figure 3.4 is the same diagram as Figure 3.1, but with glial cells added to the neuron. Oligodendrocytes manufacture the myelin sheaths for nerve axons within the CNS, a function taken over by the Schwann cells in the PNS. In Figure 3.4, examine the way in which the myelin-rich plasma membrane of oligodendrocytes wraps around the axon to provide several covering layers, which determines the thickness of the myelin sheath. The more layers there are, the better the axon is insulated. One oligodendrocyte can provide the myelin sheaths for up to 50 axons. Astrocytes are present in large numbers throughout the CNS. They have numerous cytoplasmic processes, giving the cells a star-like appearance, hence their name. Look at the structures adjacent to the astrocyte processes on Figure 3.4. Many of these processes terminate on small blood vessels, usually capillaries, while others end close to the surface of neurons; astrocytes are thus able to transport material from blood vessels to neurons. Microglia are small cells scattered throughout the CNS. They have a number of short processes and can actively ingest materials and debris, a process called phagocytosis that they share with some other cell types. They are part of the macrophage system for non-specific defence by removing dead or invasive material. Ependymal cells form a thin layer lining the ventricles of the brain and the central canal of the brainstem and spinal cord that contain cerebrospinal fluid (see Section 15.4.2).
The dotted lines across the axon in Figure 3.4 indicate the point where the axon leaves the CNS and enters the PNS. Note the change in the myelinating cells at this junction from oligodendrocytes to Schwann cells. Schwann cells are the only significant glial cells in the peripheral nervous system. They provide myelin sheaths for the peripheral nerve axons in exactly the same way as oligodendrocytes do in the CNS. Even unmyelinated axons are not naked despite lacking myelin sheaths; they are surrounded by Schwann cell or oligodendrocyte cytoplasm that serves to insulate the axons from one another. Any axon longer than a few micrometres in either the CNS or PNS requires several oligodendrocytes or Schwann cells to cover it. The contributory glial cells form an axon sheath around the neuron. As illustrated in Figure 3.4, there is a gap in the myelin sheath called a node of Ranvier where the territory of one glial cell ends and another begins. You might perhaps have already wondered how tiny ions are going to get in and out of ion channels on the neuronal cell membrane if they are covered in several thick layers of myelinated glial cell membrane. The answer is that they cannot. However, they can gain access to the neuronal cell membrane at the nodes and this is where all the ion channels are concentrated on myelinated axons. The depolarization jumps along the axon from node to node. This known as saltatory conduction and is more rapid and efficient than continuous conduction. The effects of loss of myelin is outlined in Box 3.1.
Fig. 3.4 Neuroglial cells and their relationship to neurons.
3.2.4 From neuron to nerve
All neurons have the same general structure described above, but they vary greatly in their detailed size and structure. The diameters of neuronal cell bodies range from as little as 5 μm to more than 100 μm. Axons may be less than a micrometre in diameter or as thick as 20 μm; those larger than 1 μm are usually myelinated. Axons may be less than a millimetre long or approach a metre in length. The number and pattern of branching of the dendrites are particularly variable. Axon branching can also vary considerably, especially within the CNS where significant collateral branches are given off to intermediate targets.
Variations in the overall shape of neurons are related to their function. The principal components of long tracts connecting different parts of the CNS are formed from long axons arising from large cell bodies. Long processes also constitute peripheral nerves that can be seen during dissections or are represented on anatomical models. Small neurons, on the other hand, are usually involved in forming extensive local circuits which play a major part in complex neural functions.
Recognizable anatomical nerves forming parts of the PNS that you may dissect vary from thin structures, the diameter of a cotton strand, to the thickest nerve in the body, the sciatic nerve, running through the buttocks to the leg, which is about the thickness of your thumb. Each nerve contains anything from a few tens to several thousand processes. Each optic nerve supplying the eye contains about 1,000,000 processes. The individual processes forming peripheral nerves are bundled together by a sheath of connective tissue called epineurium (See Box 3.2).
It is the convention in anatomy textbooks, including this one, to colour nerves yellow in diagrams. This is not an arbitrary choice, but one intended to convey nerves in as life-like a manner as possible. Nerves appear whitish-yellow in life and in cadaveric specimens because myelin is whitish-yellow, thus giving nerves their characteristic hue. Nerves are greyer in tone if only a few neurons in a nerve bundle are myelinated.
Processes form the major part of peripheral nerves. The cell bodies from which these processes extend are not scattered at random, but grouped together into structures called ganglia which produce a swelling on the nerve because the cell bodies are larger than the processes. Their exact anatomical location will be described in Section 3.3.
In the CNS, cell bodies, their associated dendrites, and synapses are similarly grouped together, but such a collection is known as a nucleus. These components of neurons lack myelin sheaths so nuclei have a greyish appearance and hence are known as grey matter. Grey matter is the site of synapses, therefore the place where information passing along chains of neurons can be modified. As well as forming nuclei at various places in the brain, grey matter also forms the rim of the brain, the cerebral cortex, where various higher functions are processed. Areas of grey matter are connected by axons. These interconnecting tracts appear white due to the presence of the myelin sheaths enclosing the axons, hence they are called white matter.
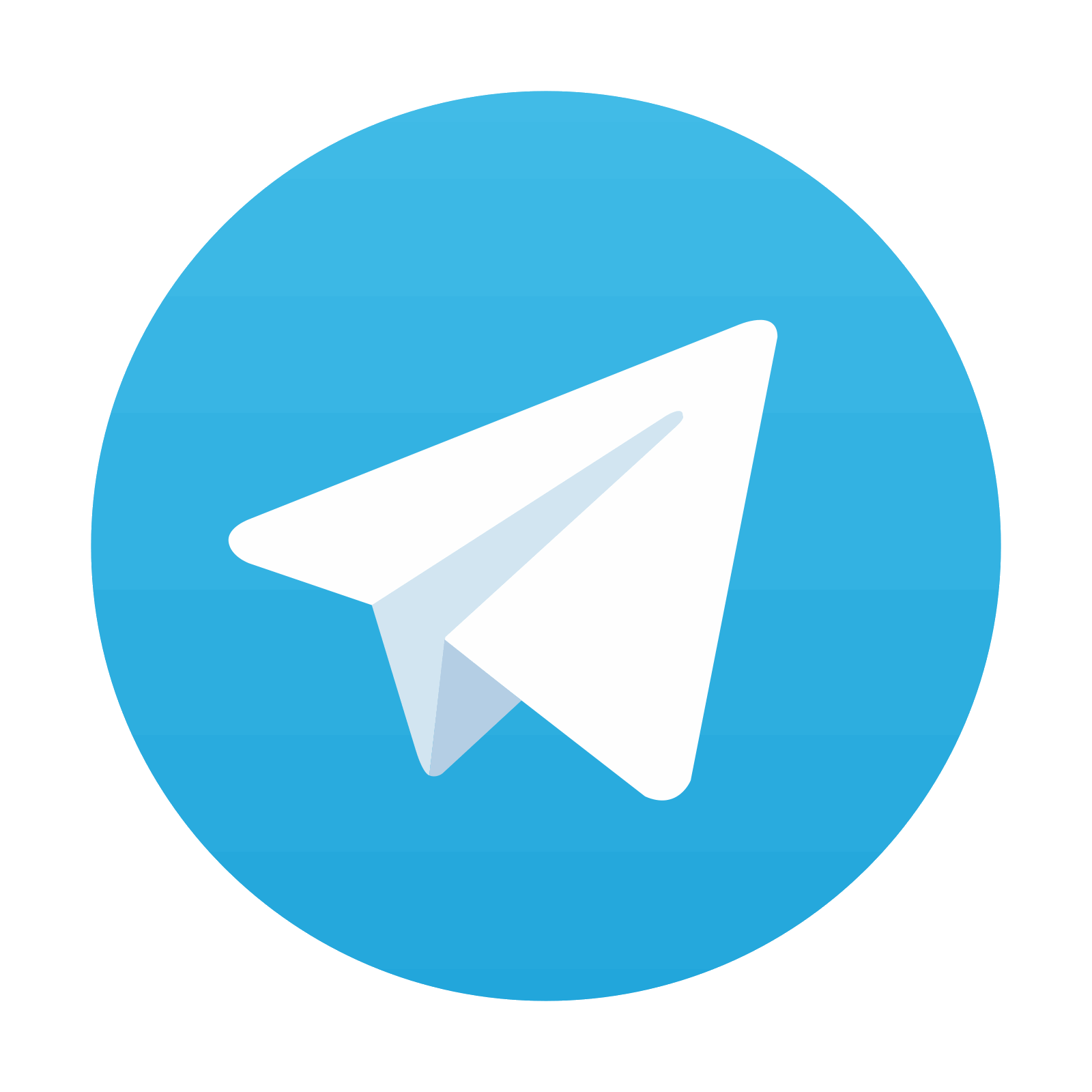
Stay updated, free dental videos. Join our Telegram channel

VIDEdental - Online dental courses
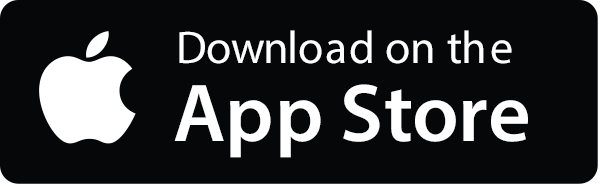
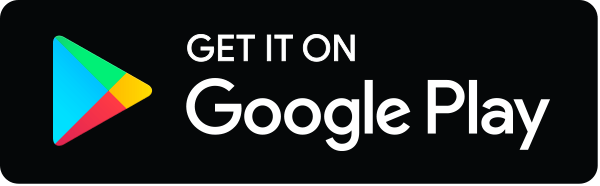