Concepts of Growth and Development
Growth: Pattern, Variability, and Timing
Figure 2-1 illustrates the change in overall body proportions that occurs during normal growth and development. In fetal life, at about the third month of intrauterine development, the head takes up almost 50% of the total body length. At this stage, the cranium is large relative to the face and represents more than half the total head. In contrast, the limbs are still rudimentary and the trunk is underdeveloped. By the time of birth, the trunk and limbs have grown faster than the head and face, so that the proportion of the entire body devoted to the head has decreased to about 30%. The overall pattern of growth thereafter follows this course, with a progressive reduction of the relative size of the head to about 12% of the adult. At birth, the legs represent about one third of the total body length, while in the adult, they represent about half. As Figure 2-1 illustrates, there is more growth of the lower limbs than the upper limbs during postnatal life. All of these changes, which are a part of the normal growth pattern, reflect the “cephalocaudal gradient of growth.” This simply means that there is an axis of increased growth extending from the head toward the feet.
Another aspect of the normal growth pattern is that not all the tissue systems of the body grow at the same rate (Figure 2-2). Obviously, as the relative decrease of head size after birth shows, the muscular and skeletal elements grow faster than the brain and central nervous system. The overall pattern of growth is a reflection of the growth of the various tissues making up the whole organism. To put it differently, one reason for gradients of growth is that different tissue systems that grow at different rates are concentrated in various parts of the body.
Even within the head and face, the cephalocaudal growth gradient strongly affects proportions and leads to changes in proportion with growth (Figure 2-3). When the skull of a newborn infant is compared proportionally with that of an adult, it is easy to see that the infant has a relatively much larger cranium and a much smaller face. This change is an important aspect of the pattern of facial growth. Not only is there a cephalocaudal gradient of growth within the body, there also is one within the face. From that perspective, it is not surprising that the mandible, being farther away from the brain, tends to grow more and later than the maxilla, which is closer.
Rather than categorizing growth as normal or abnormal, it is more useful to think in terms of deviations from the usual pattern and to express variability quantitatively. One way to do this is to evaluate a given child relative to peers on a standard growth chart (Figure 2-4). Although charts of this type are commonly used for height and weight, the growth of any part of the body can be plotted in this way. The “normal variability,” as derived from large-scale studies of groups of children, is shown by the solid lines on the graphs. An individual who stood exactly at the midpoint of the normal distribution would fall along the 50% line of the graph. One who was larger than 90% of the population would plot above the 90% line; one who was smaller than 90% of the population would plot below the 10% line.
FIGURE 2-4 A, Growth of a normal girl plotted on the chart for females. Note that this girl remained at about the 75th percentile for height and weight over this entire period of observation. B, Growth of a boy who developed a medical problem that affected growth, plotted on the male chart. Note the change in pattern (crossover of lines on the chart) between ages 10 and 11. This reflects the impact of serious illness beginning at that time, with partial recovery after age 13 but a continuing effect on growth. (Data from Hamill PVV, et al. National Center for Health Statistics, 1979; charts developed by the National Center for Health Statistics in collaboration with the National Center for Chronic Disease Prevention and Health Promotion, published May 30, 2000, revised 11/21/00.) (Charts available from http://www.cdc.gov/growthcharts/.)
These charts can be used in two ways to determine whether growth is normal or abnormal. First, the location of an individual relative to the group can be established. A general guideline is that a child who falls outside the range of 97% of the population should receive special study before being accepted as just an extreme of the normal population. Second and perhaps more importantly, growth charts can be used to follow a child over time to evaluate whether there is an unexpected change in growth pattern. Pattern implies predictability. For the growth charts, this means that a child’s growth should plot along the same percentile line at all ages. If the percentile position of an individual relative to his or her peer group changes, especially if there is a marked change (see Figure 2-4, B), the clinician should suspect some growth abnormality and should investigate further. Inevitably, there is a gray area at the extremes of normal variations, at which it is difficult to determine if growth is normal.
Variations in growth and development because of timing are particularly evident in human adolescence. Some children grow rapidly and mature early, completing their growth quickly and thereby appearing on the high side of developmental charts until their growth ceases and their contemporaries begin to catch up. Others grow and develop slowly and so appear to be behind, even though, given time, they will catch up with and even surpass children who once were larger. All children undergo a spurt of growth at adolescence, which can be seen more clearly by plotting change in height or weight (Figure 2-5), but the growth spurt occurs at different times in different individuals.
Growth effects because of timing variation can be seen particularly clearly in girls, in whom the onset of menstruation (menarche) gives an excellent indicator of the arrival of sexual maturity. Sexual maturation is accompanied by a spurt in growth. When the growth velocity curves for early-, average-, and late-maturing girls are compared in Figure 2-6, the marked differences in size between these girls during growth are apparent. At age 11, the early-maturing girl is already past the peak of her adolescent growth spurt, whereas the late-maturing girl has not even begun to grow rapidly. This sort of timing variation occurs in many aspects of both growth and development and can be an important contributor to variability.
Although age is usually measured chronologically as the amount of time since birth or conception, it is also possible to measure age biologically, in terms of progress toward various developmental markers or stages. Timing variability can be reduced by using developmental age rather than chronologic age as an expression of an individual’s growth status. For instance, if data for gain in height for girls are replotted, using menarche as a reference time point (Figure 2-7), it is apparent that girls who mature early, average, or late really follow a very similar growth pattern. This graph substitutes stage of sexual development for chronologic time to produce a biologic time scale and shows that the pattern is expressed at different times chronologically but not at different times physiologically. The effectiveness of biologic or developmental age in reducing timing variability makes this approach useful in evaluating a child’s growth status.
Methods for Studying Physical Growth
Measurement Approaches
Craniometry: The first of the measurement approaches for studying growth, with which the science of physical anthropology began, is craniometry, based on measurements of skulls found among human skeletal remains. Craniometry was originally used to study the Neanderthal and Cro-Magnon peoples whose skulls were found in European caves in the eighteenth and nineteenth centuries. From such skeletal material, it has been possible to piece together a great deal of knowledge about extinct populations and to get some idea of their pattern of growth by comparing one skull with another. Craniometry has the advantage that rather precise measurements can be made on dry skulls; it has the important disadvantage for growth studies that, by necessity, all these growth data must be cross-sectional. Cross-sectional means that although different ages are represented in the population, the same individual can be measured at only one point in time.
Anthropometry: It is also possible to measure skeletal dimensions on living individuals. In this technique, called anthropometry, various landmarks established in studies of dry skulls are measured in living individuals simply by using soft tissue points overlying these bony landmarks. For example, it is possible to measure the length of the cranium from a point at the bridge of the nose to a point at the greatest convexity of the rear of the skull. This measurement can be made on either a dried skull or a living individual, but results would be different because of the soft tissue thickness overlying both landmarks. Although the soft tissue introduces variation, anthropometry does make it possible to follow the growth of an individual directly, making the same measurements repeatedly at different times. This produces longitudinal data: repeated measures of the same individual. In recent years, Farkas’ anthropometric studies have provided valuable new data for human facial proportions and their changes over time.1
Cephalometric Radiology: The third measurement technique, cephalometric radiology, is of considerable importance not only in the study of growth but also in clinical evaluation of orthodontic patients. The technique depends on precisely orienting the head before making a radiograph, with equally precise control of magnification. This approach can combine the advantages of craniometry and anthropometry. It allows a direct measurement of bony skeletal dimensions, since the bone can be seen through the soft tissue covering in a radiograph, but it also allows the same individual to be followed over time. Growth studies are done by superimposing a tracing or digital model of a later cephalogram on an earlier one, so that the changes can be measured. Both the locations and amounts of growth can be observed in this way (Figure 2-8). Cephalometric superimposition techniques are described in detail in Chapter 6.
FIGURE 2-8 A, A cephalometric radiograph merits this name because of the use of a head positioning device to provide precise orientation of the head. This means that valid comparisons can be made between external and internal dimensions in members of the same population group or that the same individual can be measured at two points in time because the head orientation is reproducible. B, This radiograph was taken in natural head position (NHP) (see Chapter 6 for a description of this head-positioning technique).
Three-Dimensional Imaging: New information now is being obtained with the application of 3-D imaging techniques. Computed axial tomography (CAT or more commonly, CT) allows 3-D reconstructions of the cranium and face, and this method has been applied for several years to plan surgical treatment for patients with facial deformities (Figure 2-9). Recently, cone beam rather than axial CT has been applied to facial scans. This significantly reduces both the radiation dose and cost. Cone beam CT (CBCT) allows scans of patients with radiation exposure that is much closer to the dose from cephalograms. Superimposition of 3-D images is much more difficult than the superimpositions used with 2-D cephalometric radiographs, but methods developed recently are overcoming this difficulty (Figure 2-10).2 Magnetic resonance imaging (MRI) also provides 3-D images that can be useful in studies of growth, with the advantage that there is no radiation exposure with this technique. This method already has been applied to analysis of the growth changes produced by functional appliances.3 Three-dimensional photography now makes possible much more accurate measurements of facial soft tissue dimensions and changes (Figure 2-11).4 A more detailed examination of 3-D changes in growing patients almost surely will add to current knowledge of growth patterns in the near future.
Analysis of Measurement Data
Both anthropometric and cephalometric data can be expressed cross-sectionally rather than longitudinally. Obviously, it would be much easier and quicker to do a cross-sectional study, gathering data once for any individual and including subjects of different ages, rather than spending many years on a study in which the same individuals are measured repeatedly. For this reason, most studies are cross-sectional. When this approach is used, however, variability within the sample can conceal details of the growth pattern, particularly when there is no correction for timing variation (Figure 2-12). Fluctuations in the growth curve that may occur for nearly every individual would be seen in a cross-sectional study only if they occurred at the same time for each person, which is unlikely. Longitudinal studies are efficient in the sense that a great deal of information can be gained from a relatively small number of subjects, fewer than would be needed in a cross-sectional study. In addition, the longitudinal data highlight individual variations, particularly variations caused by timing effects.
Measurement data can be presented graphically in a number of different ways, and frequently, it is possible to clarify growth changes by varying the method of display. For example, we have already seen that growth data can be shown either by plotting the size attained as a function of age, which is called a “distance” curve, or as a “velocity” curve, showing not the total length but the increment added each year (see Figure 2-5). Changes in the rate of growth are much more easily seen in a velocity curve.
Various other mathematical transformations can be used with growth data to make them easier to understand. For instance, the growth in weight of any embryo at an early stage follows a logarithmic or exponential curve because the growth is based on division of cells; the more cells there are, the more cell divisions can occur. If the same data are plotted using the logarithm of the weight, a straight-line plot is attained (Figure 2-13). This demonstrates that the rate of multiplication for cells in the embryo is remaining more or less constant.
More complex mathematical transformations were used many years ago by D’Arcy Thompson5 to reveal similarities in proportions and growth changes that had not previously been suspected (Figure 2-14). To correctly interpret data after mathematical transformation, it is important to understand how the data were transformed, but the approach is a powerful one in clarifying growth concepts. Thompson’s classic presentation remains stimulating reading.
Experimental Approaches
Much has been learned about skeletal growth using the technique called vital staining, in which dyes that stain mineralizing tissues (or occasionally, soft tissues) are injected into an animal. These dyes remain in the bones and teeth and can be detected later after sacrifice of the animal. This method was originated by the great English anatomist John Hunter in the eighteenth century. Hunter observed that the bones of pigs that occasionally were fed textile waste were often stained in an interesting way. He discovered that the active agent was a dye called alizarin, which still is used for vital staining studies. Alizarin reacts strongly with calcium at sites where bone calcification is occurring. Since these are the sites of active skeletal growth, the dye marks the locations at which active growth was occurring when it was injected. Bone remodels rapidly, and areas from which bone is being removed also can be identified by the fact that vital stained material has been removed from these locations (Figure 2-15). Highly detailed vital staining studies of bony changes in craniofacial development in experimental animals, from work done at the National Institute of Dental Research, are available.6
Although studies using vital stains are not possible in humans, vital staining can occur. Many children born in the late 1950s and early 1960s were treated for recurrent infections with the antibiotic tetracycline. It was discovered too late that tetracycline is an excellent vital stain that binds to calcium at growth sites in the same way as alizarin. The discoloration of incisor teeth that results from tetracycline given when the teeth are mineralizing has been an esthetic disaster for some individuals (Figure 2-16). Although this should not occur now, it still is seen occasionally.
With the development of radioactive tracers, it has become possible to use almost any radioactively labeled metabolite that becomes incorporated into the tissues as a sort of vital stain. The location is detected by the weak radioactivity given off at the site where the material was incorporated. The gamma-emitting isotope 99mTc can be used to detect areas of rapid bone growth in humans, but these images are more useful in diagnosis of localized growth problems (see Chapter 19) than for studies of growth patterns. For most studies of growth, radioactively labeled materials in the tissues of experimental animals are detected by the technique of autoradiography, in which a film emulsion is placed over a thin section of tissue containing the isotope and then is exposed in the dark by the radiation. After the film is developed, the location of the radiation that indicates where growth is occurring can be observed by looking at the tissue section through the film (Figure 2-17).
Implant Radiography
Another experimental method applicable to studies of humans is implant radiography. In this technique, inert metal pins are placed in bones anywhere in the skeleton, including the face and jaws. These metal pins are well tolerated by the skeleton, become permanently incorporated into the bone without causing any problems, and are easily visualized on a cephalogram (Figure 2-18). If they are placed in the jaws, a considerable increase in the accuracy of a longitudinal cephalometric analysis of growth pattern can be achieved. This method of study was developed by Professor Arne Björk and coworkers at the Royal Dental College in Copenhagen, Denmark, and was used extensively by workers there (see Chapter 4). It provided important new information about the growth pattern of the jaws. Before radiographic studies using implants, the extent of remodeling changes in the contours of the jaw bones was underestimated, and the rotational pattern of jaw growth described in Chapter 4 was not appreciated.
Genetic Influences on Growth
Rapid advances in molecular genetics are providing new information about growth and its control. For example, homeobox Msx genes, which are known to be critically important in the establishment of body plan, pattern formation, and morphogenesis, have been found to be expressed differentially in growth of the mandible. Msx1 is expressed in basal bone but not in the alveolar process, while Msx2 is strongly expressed there.7 It is known now that a decrease in Hedgehog pathway activity causes holoprosencephaly (failure of the nose to develop) and hypotelorism and that excessive activity due to truncating primary cilia on cranial neural crest cells causes hypertelorism and frontonasal dysplasia.8 It also has been shown recently that hedgehog signaling acts at two distinct steps in disk morphogenesis: condyle initiation and disc-condyle separation during the formation of the temporomandibular (TM) joint.9 The proper functioning of families of growth factors and their cognate receptors is essential in regulating embryonic processes of cell growth and organ development, as well as a myriad of postnatal processes that include growth, wound healing, bone remodeling, and homeostasis.
An exciting prospect is a better understanding of how patients with orthodontic problems that are known to have a genetic component (Class III malocclusion being the best example) will respond to treatment. Chromosomal loci associated with Class III malocclusion have been identified.10 It is clear that there are multiple subtypes of Class III, and a necessary first step is better characterization of these phenotypes. Establishing phenotypic markers (distinct clinical characteristics) makes it possible to establish definitive correlations with modes of inheritance and is necessary for linkage studies that will clarify the genetic basis for the problem. At this point, the mutation leading to primary failure of eruption (PFE) has been identified,11 and for the first time, an orthodontic problem can be diagnosed from a sample of blood or saliva. It is likely that in the future, genetic screening of blood or other tissue samples will be used to identify patients with orthodontic problems who are likely to respond well or poorly to specific treatment modalities, in the same way that the likely response to drug therapies already is being determined.12
Experiments that clarify how growth is controlled at the cellular level offer exciting prospects for better control of growth in the future. It is estimated that about two-thirds of the 25,000 human genes play a role in craniofacial development, so complex patterns of genetic activity obviously are involved, and complex genetic interactions interact with external influences on growth. It is unlikely that genetic analysis will ever be applicable to planning treatment for the majority of orthodontic problems, but it could yield valuable information about the best approach to some of the most difficult skeletal malocclusions and perhaps the application of gene therapy to growth problems.13
The Nature of Skeletal Growth
Figure 2-19 shows the cartilaginous or chondrocranium at 8 and 12 weeks of intrauterine development. Cartilaginous skeletal development occurs most rapidly during the third month of intrauterine life. A continuous plate of cartilage extends from the nasal capsule posteriorly all the way to the foramen magnum at the base of the skull. It must be kept in mind that cartilage is a nearly avascular tissue whose internal cells are supplied by diffusion through the outer layers. This means, of course, that the cartilage must be thin. At early stages in development, the extremely small size of the embryo makes a chondroskeleton feasible, but with further growth, such an arrangement is no longer possible without an internal blood supply.
During the fourth month in utero, there is an ingrowth of blood vascular elements into various points of the chondrocranium (and the other parts of the early cartilaginous skeleton). These areas become centers of ossification, at which cartilage is transformed into bone in the process called endochondral ossification, and islands of bone appear in the sea of surrounding cartilage (see Figure 2-19, B). The cartilage continues to grow rapidly but is replaced by bone with equal rapidity. The result is that the amount of bone increases rapidly and the relative (but not the absolute) amount of cartilage decreases. Eventually, the old chondrocranium is represented only by small areas of cartilage interposed between large sections of bone, which assume the characteristic form of the ethmoid, sphenoid, and basioccipital bones. Growth at these cartilaginous connections between the skeletal bones is similar to growth in the limbs.
In the long bones of the extremities, areas of ossification appear in the center of the bones and at the ends, ultimately producing a central shaft called the diaphysis and a bony cap on each end called the epiphysis. Between the epiphysis and diaphysis is a remaining area of uncalcified cartilage called the epiphyseal plate (Figure 2-20, A). The epiphyseal plate cartilage of the long bones is a major center for their growth, and in fact, this cartilage is responsible for almost all growth in length of these bones. The periosteum on the surfaces of the bones also plays an important role in adding to thickness and in reshaping the external contours.
Endochondral ossification also occurs at the mandibular condyle, which superficially looks like half an epiphyseal plate (Figure 2-20, B and C). As we will see, however, the cartilage of the condyle does not behave like an epiphyseal plate—and the difference is important in understanding mandibular growth.
Not all bones of the adult skeleton were represented in the embryonic cartilaginous model, and it is possible for bone to form by secretion of bone matrix directly within connective tissues, without any intermediate formation of cartilage. Bone formation of this type is called intramembranous ossification. This type of bone formation occurs in the cranial vault and both jaws (Figure 2-21).
Early in embryonic life, the mandible of higher animals develops in the same area as the cartilage of the first pharyngeal arch—Meckel’s cartilage. It would seem that the mandible should be a bony replacement for this cartilage in the same way that the sphenoid bone beneath the brain replaces the cartilage in that area. In fact, development of the mandible begins as a condensation of mesenchyme just lateral to Meckel’s cartilage and proceeds entirely by intramembranous bone formation (Figure 2-22). Meckel’s cartilage disintegrates and largely disappears as the bony mandible develops. Remnants of this cartilage are transformed into a portion of two of the small bones that form the conductive ossicles of the middle ear but not into a significant part of the mandible. Its perichondrium persists as the sphenomandibular ligament. The condylar cartilage develops initially as an independent secondary cartilage, which is separated by a considerable gap from the body of the mandible (Figure 2-23). Early in fetal life, it fuses with the developing mandibular ramus.
Whatever the location for intramembranous bone formation, interstitial growth within the mineralized mass is impossible, and the bone must be formed entirely by apposition of new bone to free surfaces. Its shape can be changed through removal (resorption) of bone in one area and addition (apposition) of bone in another (see Figure 2-15). This balance of apposition and resorption, with new bone being formed in some areas while old bone is removed in others, is an essential component of the growth process. The formation of new bone from a cartilaginous predecessor or direct bone formation within mesenchyme often is referred to as modeling; changes in the shape of this new bone due to resorption and replacement are referred to as remodeling. Keeping this distinction in mind can make it easier to understand the following sections of this chapter.
Sites and Types of Growth in the Craniofacial Complex
Cranial Vault
At birth, the flat bones of the skull are rather widely separated by loose connective tissues (Figure 2-24). These open spaces, the fontanelles, allow a considerable amount of deformation of the skull at birth. This is important in allowing the relatively large head to pass through the birth canal (see Chapter 3 for more detail). After birth, apposition of bone along the edges of the fontanelles eliminates these open spaces fairly/>
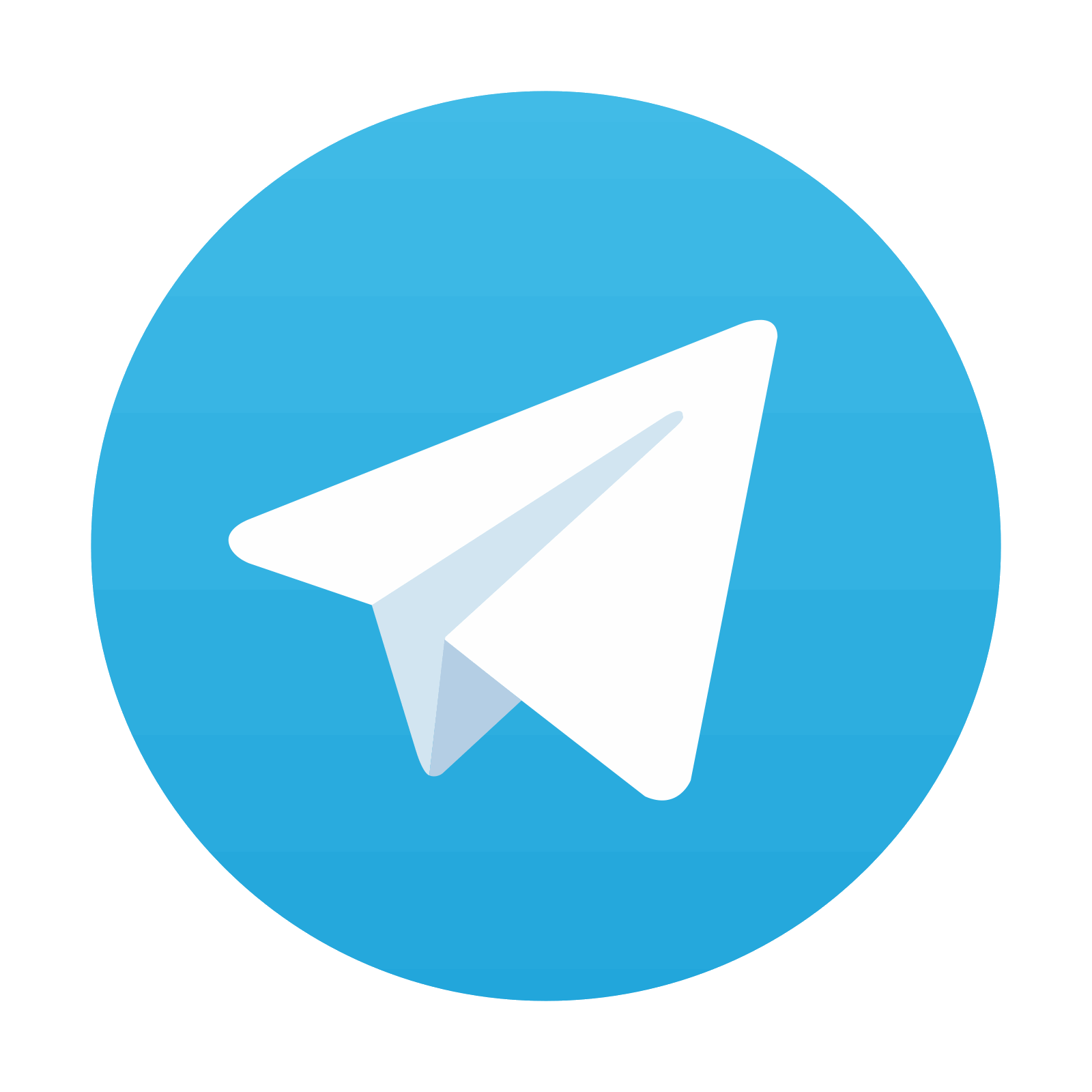
Stay updated, free dental videos. Join our Telegram channel

VIDEdental - Online dental courses
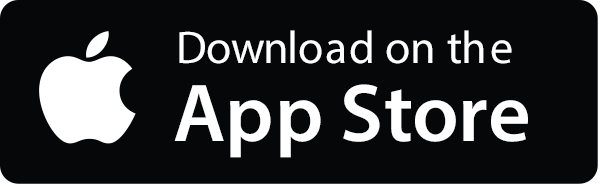
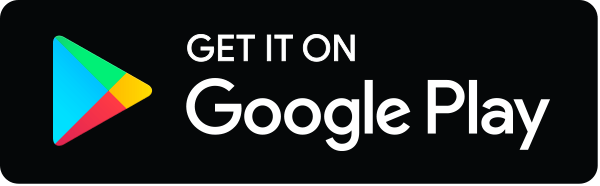