CHAPTER 11 Introduction to Central Nervous System Drugs*
Drugs that alter synaptic function are likely to have considerable impact on neuronal activity. An understanding of these fundamental mechanisms permits appreciation of the therapeutic actions and side-effect profiles of many of the central nervous system (CNS) drugs in the brain and in the spinal cord. The interested student is encouraged to seek several excellent reviews in this area.23,30 The pharmacology of specific CNS drugs is discussed in greater detail in Chapters 12 through 21 and Chapter 23.
INVESTIGATION OF THE BRAIN
In the last two decades the pace of discovery has accelerated considerably. Experiments that previously took years can often be performed in days. Genomic approaches allow tens of thousands of biochemical reactions to be studied simultaneously. A key development was the polymerase chain reaction by Mullis at Cetus Corp. Mullis shared the Nobel Prize in Chemistry in 1993 for his part in the invention. The invention of the gene microarray is another technology that is important in this respect.32 The data from these experiments are analyzed using computers rather than manually. These techniques are beginning to reveal how networks of genes, amino acids, proteins, lipids, sugars, and other chemicals interact to mediate the various functions of the brain. A genomic study of tissue from a patient with epilepsy revealed that the seizures were associated with excess glutamate release from the astrocytes.27
Future investigations of the brain may reveal that many chronic brain disorders result from tissue inflammation or breakdown, rather than dysregulation of a particular neurotransmitter. This hypothesis seems to be true in the case of schizophrenia and Alzheimer’s disease.32,37 In 2008, the Nobel Prize in Chemistry was awarded to Shimomura, Chalfie, and Tsien for the development of the green fluorescent protein technique, which has facilitated understanding of genetic modifications in dense tissue such as the brain. Other powerful techniques applicable to brain research are continually being developed.
The CNS integrates sensory information from the external and internal environments; maintains homeostasis through visceral and somatic secretory and motor activity; and generates memory, thoughts, and emotions. Many common diseases have their origins in CNS dysfunction, including Alzheimer’s disease, epilepsy, stroke, anxiety, psychoses, movement disorders, mental impairment, and some forms of chronic pain. In addition, the therapeutic effects or side effects of many drugs arise from alterations in CNS activity. Approximately 20% of the most frequently prescribed medications have their principal sites of action within the CNS (e.g., opioid-containing analgesics, benzodiazepines such as alprazolam, antidepressants such as sertraline and fluoxetine, and sleep aids such as zolpidem), and it is virtually certain that every practicing dentist will perform dental treatment on patients taking these drugs. This chapter reviews the anatomic, cellular, and biochemical organization of the CNS from the perspective of drug actions in the CNS. Table 11-1 lists representative drugs that act on the CNS to produce their therapeutic effects.
ANATOMIC ORGANIZATION OF THE CENTRAL NERVOUS SYSTEM
Several excellent texts are available that provide comprehensive descriptions of the organizational structure and pharmacology of the CNS.13,23,30,31 This section reviews only the key structural elements of the CNS most pertinent to understanding drug actions and effects.
Cerebral Cortex
Anatomy
A primary organizational feature of the cerebral cortex is its arrangement as a series of densely packed columns of interconnected cells. The columnar organization of the cerebral cortex is probably a major factor in the integration of neural activity. Each column is approximately 0.5 mm to 1 mm in diameter and includes 10,000 to 50,000 interconnected neurons. The classic studies by Penfield and Rasmussen40 determined the somatic representation of the human body surface on the sensory cortex (the “sensory homunculus”). These studies indicated that approximately 75% of the sensory cortex processes afferent input from orofacial structures, including the lips, jaws, tongue, and teeth. This predominant cerebral processing of orofacial sensation may contribute to the aversive anxiety that many patients have during the course of dental care.
Pharmacology
Drugs that alter cerebral cortical activity include general anesthetics, antianxiety drugs, sedative-hypnotics, anticonvulsants, antidepressants, and antipsychotics. As detailed subsequently (and in later chapters), the sites of action and the precise biochemical mechanisms for many of these drugs are still incompletely understood. The clinical consequence of a reduction in cortical activity is generally sedation or unconsciousness, however. Administration of the inhalation anesthetic halothane reduces cerebral activity in the frontal cortex during the induction of general anesthesia.14 Opioids produce analgesia in part by binding to the cingulate and insular cortex, which play a role in registering the aversive qualities of stimuli.5
Limbic System
Anatomy
Another major organizational component of the CNS is the limbic system. It is composed of the amygdala, septum, hippocampus, hypothalamus, olfactory lobes, basal ganglia, and portions of the thalamus. These interrelated structures act to coordinate affective (i.e., emotional) sensations with motor, visceral, and endocrine functions. Many of these structures are reciprocally connected with the cerebral cortex, and some are arranged in loops. Such loops integrate the functions of different parts of the brain but can also play a role in brain disease when disturbances develop along a loop. These loops may play a role in Parkinson’s disease and drug abuse. In addition, many behavioral functions ascribed to the limbic system are linked functionally to the reticular formation. Hyperexcitation of the amygdala has been associated with panic attacks.41 The hypothalamus is important for endocrine function, but it is also an important regulator of cyclic functions such as waking and sleeping, monthly ovulatory function, and longer cycles such as yearly hibernation in animals. The study of these periodic phenomena is termed chronobiology.
Pharmacology
Many drugs act in part by modifying the activity of the limbic system. Benzodiazepines act at several discrete sites within this system to potentiate the effects of the neurotransmitter γ-aminobutyric acid (GABA), resulting in a reduction of anxiety and the development of sedation (see Table 11-1).50 Benzodiazepines also reduce seizure activity. Endogenous ligands for the benzodiazepine receptor may be involved in the pathogenesis of epilepsy because epileptic patients have a significant reduction in benzodiazepine receptors in the cortex and limbic system.46 If administered in excess, local anesthetics such as lidocaine and the antibiotic penicillin may induce seizure activity.
A major hypothesis for some forms of mental dysfunction (e.g., schizophrenia) proposes an excess in dopaminergic activity. Several antipsychotic drugs are dopamine receptor antagonists and are thought to act at various sites in the limbic system and reticular formation. The clinical consequence of dopamine receptor blockade in these patients is the amelioration of psychotic behavior. Parkinson’s disease is associated with a chronic reduction of dopamine activity in the basal ganglia complex.55 This disease is commonly managed by the administration of drugs such as levodopa (l-dihydroxyphenylalanine, the amino acid precursor to dopamine) that increase dopamine activity. Dopamine is an important neurotransmitter in the brain reward circuitry located in the basal ganglia, and it plays a role in the development of drug dependence to cocaine and amphetamines and indirectly to other drugs.12,19
Midbrain and Brainstem
Anatomy
The midbrain and brainstem regions consist of the mesencephalon, pons, medulla, reticular activating system, and most of the cranial nerve nuclei, including the trigeminal nuclei. This region processes sensory information from the viscera, coordinates visceral (i.e., cardiovascular, pulmonary, and gastrointestinal) systems, and integrates various reflexes (e.g., swallowing and vomiting). In addition, the reticular activating system is implicated in the maintenance of arousal and development of sleep. Damage to small areas of the brainstem can be lethal if they interfere with cardiovascular or respiratory control. The reticular activating system is sensitive to many drugs, including most CNS depressants.7
Spinal Cord
The spinal cord is involved with the processing and modulation of general sensory information (e.g., touch, heat, cold, pressure, and pain), somatic motor activity, and skeletal and visceral reflexes. Numerous drugs are thought to activate spinal cord mechanisms. Opioids produce analgesia in part by stimulating receptors located in the spinal dorsal horn. (An analogous site of action for opioid inhibition of trigeminal pain involves interaction with receptors located in the medullary dorsal horn, as mentioned in Chapter 20.) This site of action is the basis for the administration of opioids through epidural catheters to elicit spinal analgesia. In addition, the epidural administration of local anesthetics such as bupivacaine is commonly used for the production of regional anesthesia in surgical and obstetric procedures. More recent studies suggest that nonsteroidal anti-inflammatory drugs produce analgesia after intrathecal injection, suggesting that these drugs have both central and peripheral sites of action.
Blood-Brain Barrier
The blood-brain barrier is not completely developed at the time of birth, and many drugs administered to neonates achieve greater concentrations in the CNS than occur in older children or adults.29 Numerous other conditions can produce a temporary breakdown of the blood-brain barrier, which can increase the penetration of drugs such as morphine and antibiotics into the brain. Some of these conditions include hypertension, inflammation, hypercapnia, osmotic stress, multiple sclerosis, and hyperthermia.
Brain Imaging
Tomographic reconstruction was subsequently extended to other forms of detectable energy. Chemicals labeled with positron emitting isotopes can be visualized based on the geometry of their emitted radiation, which permits back calculation of the point of origin. This technique is referred to as positron emission tomography (PET); a less expensive variant is single-photon emitted computed tomography (SPECT). Most of the energy in the brain is needed for the Na+,K+-ATPase pumps that maintain the membrane potential. Monitoring glucose, oxygen, or adenosine triphosphate (ATP) permits functional assessment of the activity in the brain.49 An early functional PET scan used fluorodeoxyglucose to follow glucose use.4 Labeling compounds that bind selectively to known proteins, such as the dopamine uptake transporter, permitted the visualization of its brain binding sites.55 In Parkinson’s disease, these techniques show a loss of dopamine uptake in the basal ganglia. Although these results were exciting, they also were limited to fuzzy images.
Functional MRI and DTI are primarily experimental techniques at this time but are likely to move into the clinic.33 One problem with these techniques is temporal resolution; they require a few seconds to get enough information for an image. Magnetoencephalography is a new technique with a temporal resolution of 2 msec and may be useful when faster techniques are needed.
Structural Features of the Neuron
Communication within the CNS incorporates digital and analog encoding.13,30 The digital encoding consists of the frequency of action potential depolarizations that are conveyed along the plasma membrane of the axon. Axon potentials are termed all or none, meaning that when the threshold for triggering an action potential is reached, the cell generates a complete action potential, whose maximal voltage is consistent for its travel down the axon. The major pharmacologic intervention for this form of signal encoding is the use of drugs that block ion channels, preventing the initiation and conduction of action potentials. The blockade of Na+ channels by local anesthetics is a primary example. The CNS effect is the basis for many of the toxic effects of local anesthetics (e.g., sedation, convulsions).
Uncharacteristic of a pyramidal neuron in the brain, the primary afferent neuron releases biologically active compounds at both ends of the cell—that is, within the brainstem but also at the sites of sensory input.43 In the brainstem, these chemicals (glutamate, substance P, calcitonin gene–related peptide) are considered neurotransmitters. These substances are also transported to the site of an injury, however, and mediate inflammation and chemotaxis of inflammatory cells. Similarly, the transient receptor potential vanilloid 1 receptor, a nonspecific ion channel that mediates the burning pain associated with hot chili peppers,54 is present in the dorsal root ganglia and transported to the peripheral nerve ending and the spinal cord.26
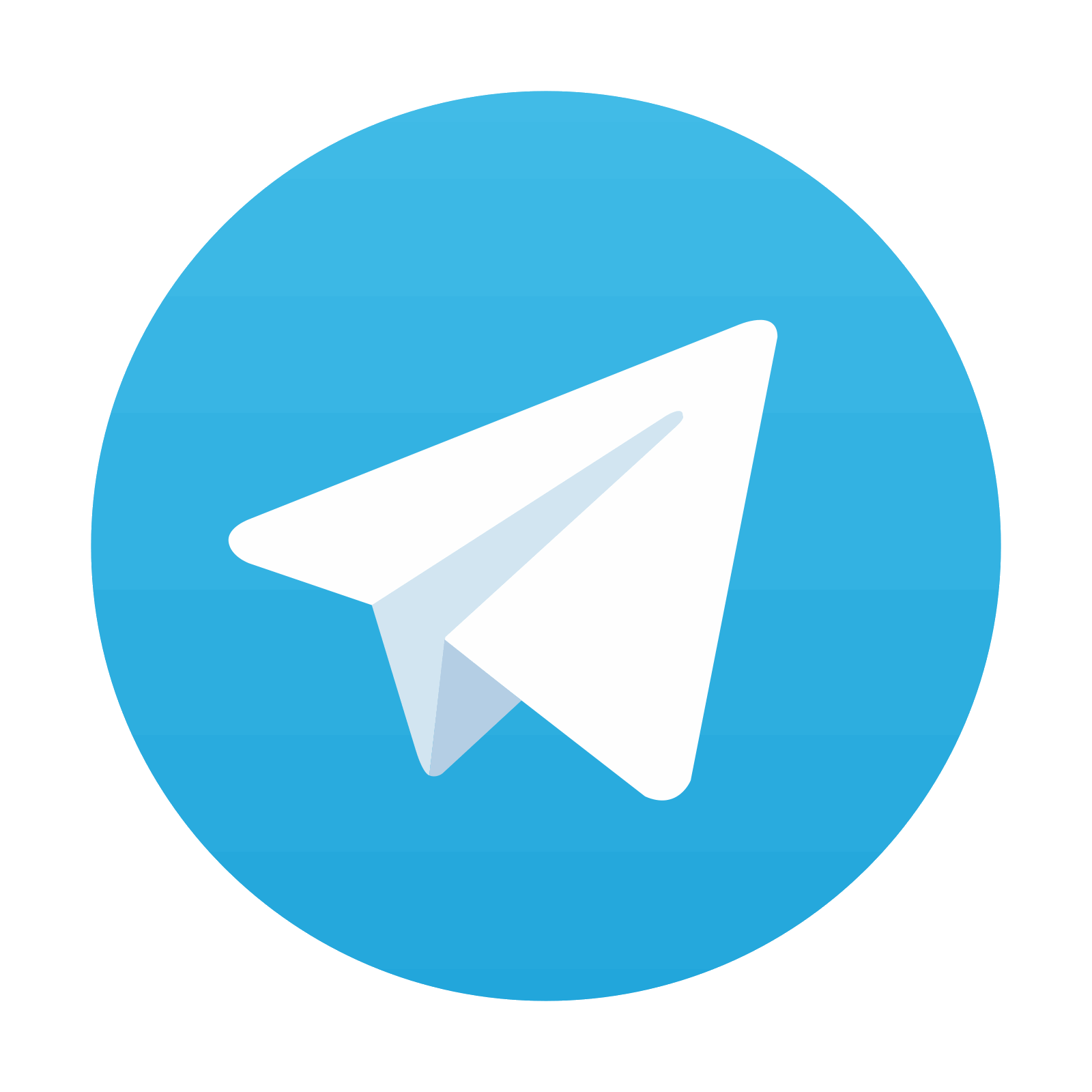
Stay updated, free dental videos. Join our Telegram channel

VIDEdental - Online dental courses
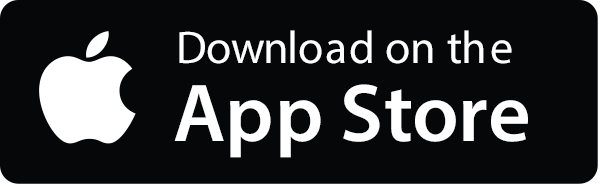
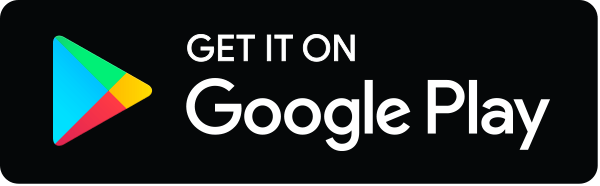