3
Treatment of Immature Teeth with Pulp Necrosis
Paul R. Cooper1, Henry F. Duncan2, Matthias Widbiller3, and Kerstin M. Galler3
1 Sir John Walsh Research Institute, Department of Oral Sciences, Faculty of Dentistry, University of Otago, Dunedin, New Zealand
2 Division of Restorative Dentistry and Periodontology, Trinity College Dublin, Dublin Dental University Hospital, Dublin, Ireland
3 Department of Conservative Dentistry and Periodontology, University Hospital Regensburg, Regensburg, Germany
3.1 Introduction
In immature teeth, if left untreated, carious lesions, traumatic injuries, and developmental anomalies (dens invaginatus, dens evaginatus) may lead to pulpitis, pulp necrosis, and eventually apical periodontitis. Once the crown of an erupting tooth appears in the oral cavity, it will still take approximately three years before root formation is complete. Depending on the stage of root development, conventional root canal treatment (RCT) may not be possible due to several anatomical characteristics: a wide‐open apical foramen with blunderbuss shape, thin root canal walls, and an unfavourable crown‐root ratio, all of which increase the risk of fracture. Whereas a vital pulp should be preserved and receive vital pulp treatment (VPT) [1], therapies for immature teeth with pulp necrosis aim at resolving signs and symptoms of infection, and at the long‐term preservation of affected teeth.
Two treatment modalities are currently considered suitable for immature teeth with pulp necrosis: an apical plug with mineral trioxide aggregate (MTA) and revitalization. Placement of MTA or another hydraulic calcium silicate cement (HCSC) in contact with the periapical tissue enables osseous healing and induces mineralized tissue formation adjacent to the material [2–5]. The core procedural detail for revitalization is the induction of bleeding into the root canal, where the blood clot serves as a starting point for healing. Thus, revitalization offers the potential to enable root lengthening and thickening, and may thus be advantageous in stabilizing the thin dentinal walls of immature teeth, reducing the risk of fracture.
This idea of provocation of bleeding into the root canal dates back to the 1960s, when Nygaard‐Østby conducted studies in animals and patients to show that new tissue formation was possible after allowing the root canal to fill with blood [6]. In dental traumatology, it was observed early on that, following avulsion, immature teeth could present with a newly formed vascular network throughout the canal only 30 days after replantation [7]. The term ‘revascularization’ was thus used for the first cases published on the provocation of bleeding into immature teeth with pulp necrosis. As expectations grew to include the idea of inducing regeneration with this protocol, the term ‘regenerative endodontic procedure’ was coined [8]. ‘Revitalization’ reflects the formation of new tissue inside the root canal without specifying the type [9], whilst the term ‘guided endodontic repair’ takes into account that the procedure is controlled and aimed at tissue repair and healing [10].
The radiographically observed response to revitalization shows a wide range, including unaltered apical anatomy, apical closure, narrowing of the root canal, increased root length and thickness, root canal obliteration, and completion of root formation [11–15]. A multitude of parameters influence the outcome after revitalization. Non‐operator‐dependent factors include the patient’s individual anatomy, the stage of root development, the pathosis, the type of injury and tissue damage, the extent of infection and inflammation, and the patient’s healing capacity. Operator‐dependent factors, meanwhile, include the determination of indication, the procedural details (particularly the use of certain agents and materials, concentrations, and durations of action), and, importantly, the disinfection protocol. Whilst the clinician must take the non‐operator‐dependent factors into account and make a reasonable decision regarding the choice of treatment in each individual case, these factors cannot be altered. However, the use of biomaterials during the procedure is the operator’s choice and requires an in‐depth knowledge of the agents and materials in question, their composition, and their effects on cells and tissues. In order to better understand the interactions of materials and tissue reactions, it is indispensable to have a deep knowledge of the healing process, inflammation, growth factor signalling, and potential epigenetic influences – all of which will be discussed in this chapter.
Whereas current clinical protocols induce tissue repair, true regeneration of the dentine–pulp complex is subject to continuous research efforts. Tissue engineering approaches for dental pulp regeneration have been applied in cell‐based and cell‐free systems, and proof of principle exists that dental pulp regeneration is possible. The development of increasingly sophisticated scaffold materials and the use of dentine‐derived growth factors may enable us to implement such concepts in the clinic in the near future. Again, an in‐depth understanding of the procedures, materials, and tissue responses will be required to conduct successful regenerative treatment in endodontics.
3.2 Apexification and Root‐End Closure
If dental caries is allowed to proceed without remedial treatment, the microbial biofilm will advance and bacteria will invade the pulp tissue. This challenge leads to irreversible pulpits, pulp necrosis, and subsequent apical periodontitis [16]. Management of pulp necrosis in mature teeth requires RCT, but if the root formation is not complete, the combination of thin dentine walls and open apices will make completion of conventional RCT impossible [17]. Furthermore, immature teeth which have lost vitality are also more vulnerable to injury (losing the ability to sense environmental change) and more prone to root fracture compared to mature teeth [18, 19].
From a biological perspective, the treatment of nonvital immature teeth requires the same level of disinfection and removal of necrotic tissue as conventional RCT, albeit with a reduction or absence of root canal instrumentation. Traditional treatment of immature teeth is by apexification, a technique that induces an apical mineralized barrier using nonsetting calcium hydroxide (CH) over a period of a few months. During the apexification procedure, the necrotic tissue is removed via chemomechanical disinfection, whilst the canal is dried and dressed with a nonsetting CH paste placed carefully on to the tooth apex with an endodontic plugger or spiral filler in order to ensure good contact with the apical tissues. The CH is generally changed after one month, and thereafter every three months, until an apical barrier forms. This barrier is usually a poor‐quality cementum‐like tissue that can be viewed radiographically, and its presence is confirmed by gentle palpation with a paper point. The entire process takes anywhere from 6 to 18 months to complete. Once confirmed, the teeth can be filled with thermoplastic gutta‐percha. From a practical perspective, apexification is clearly time‐consuming [20] and costly for both patients and dentists, whilst the predictable creation of a mineralized barrier is hard to achieve [21]. Notably, root fracture is significantly increased with long‐term CH dressing in immature teeth [18].
As a result, root‐end closure techniques, which involve placing an HCSC matrix at the apex [22, 23], have recently been preferred over long‐term CH therapy. The use of an apical plug reduces treatment time to one or two visits, limiting the risk of loss of patient compliance and of root fracture (Figure 3.1). Although not essential, the use of magnifying loupes or an operating microscope facilitates this treatment, which (at least initially) is carried out in an identical manner to apexification using CH. An HCSC, ideally possessing a radioaopacity greater than dentine, is placed gently with an endodontic plugger or specialized MTA carrier until a thickness of 5 mm from the radiographic apex in achieved [23]. Thereafter, the tooth can be dressed with a moist cotton pellet or paper point prior to the placement of thermoplastic gutta‐percha at a subsequent visit; alternatively, it may be filled in a single visit if the HCSC sets.
Notably, treatment outcome comparisons have highlighted a similar or improved response with root‐end closure using MTA over traditional apexification techniques using CH [24, 25]. However, both these techniques are not designed to induce extension of root length or width [26, 27], and they generally have poor long‐term prognosis [28]. Therefore, strategies to either maintain the healthy pulp or stimulate the development of new biological tissue are paramount, not only to promote minimally invasive solutions, but also to retain or reinstate the capacity of the pulp to generate tertiary dentine and respond to injurious stimuli [29].

Figure 3.1 MTA root‐end closure in a necrotic immature central incisor. (a) Immature incisor with apical radiolucency and wide root canal apically. (b) MTA plug placed following noninstrumentation protocol. (c) Remainder of canal filled with thermoplastic gutta‐percha and resin‐based composite extending below the CEJ. Note the relatively thin dentinal walls, particularly in the cervical area, which predisposes the tooth to subsequent fracture.
Source: Paul Cooper, Henry F. Duncan, Matthias Widbiller, Kerstin M. Galler.
3.3 Revitalization
Within the last few years, revitalization has been established as an alternative treatment to the apical plug, and sufficient evidence is available to show that the outcomes in terms of tooth survival and success rates are similar across both treatment modalities [2].
The first case reports from the early 2000s suggested provoking bleeding into the root canal and covering the blood clot with MTA instead of inducing an apical barrier or placing an apical plug with MTA. Follow‐up in immature teeth after this procedure showed a completion of root formation where radiographically both root lengthening and root thickening were observed [15, 30]. These results sparked interest and raised the question whether pulp regeneration was possible using such an approach. More case reports, and then case series, were published. The verification of the presence of mesenchymal stem cells in the root canal led to the hypothesis that provocation of bleeding would induce an influx of stem cells from the apical papilla, which could differentiate and form new pulp and tubular dentine [31]. A more realistic evaluation of revitalization indicates that repair rather than regeneration takes place after this procedure. This is based on results from animal studies and histological analysis of human teeth following revitalization [32–34].
3.3.1 Indications
Revitalization is indicated in immature teeth with pulp necrosis, with or without a periapical lesion. Thus, this treatment is an alternative to the apical plug. Recently, it has been suggested that revitalization is beneficial in teeth at stages 1–3 of root development according to Cvek (less than half to over two‐thirds of root development with open apex), whilst at stage 4 (nearly completed root formation with open apex) either revitalization or an MTA plug can be performed [35]. Immature permanent teeth with a necrotic pulp requiring a post to ensure an adequate coronal restoration are not suitable for revitalization and should be treated with an apical plug and root canal filling.
Whereas revitalization is easier to perform from a technical perspective when compared to the placement of an apical plug, it requires high compliance of the patient, as the induction of bleeding may cause discomfort or even pain despite the anaesthesia.
3.3.2 Procedure
The European Society of Endodontology (ESE) and American Association of Endodontists (AAE) have put forward recommendations on the procedural details of revitalization therapy. Whereas minor differences can be found between the protocols, single details may not be critical for success; rather, it is the clinician’s understanding of the procedure and the response of the involved tissues and cells to manipulation that are important.
The procedure is undertaken in two visits. The first includes proper diagnostics, field isolation, preparation of an access cavity, and disinfection by 1.5–3.0% sodium hypochlorite (NaOCl) and then saline, followed by 17% ethylenediaminetetraacetic acid (EDTA). The root canal walls should be minimally or not at all instrumented. CH can be used as an intracanal medicament and a restoration, providing a tight seal is placed. Saline and EDTA may reduce the cytotoxic effects of NaOCl on the periapical tissues. Antibiotics have been used as an intracanal medicament in early case reports, case series, and clinical studies, but the routine use of antibiotic pastes (ciprofloxacin, metronidazole, and minocycline or cefuroxim) is no longer recommended due to sensitization, bacterial resistance, and limited removability. Signs and symptoms of inflammation should have receded before the next steps are executed.
During the second visit, the tooth is reopened and the canal is rinsed with 17% EDTA and dried. Note that NaOCl is no longer indicated at this point, as it may interfere with the desired effects of EDTA, namely exposure of collagen and dentine‐derived growth factors on the surface [36]. Bleeding is induced by mechanical irritation of the periapical tissues and should reach up below the cementoenamel junction (CEJ). Placement of a collagenous material is optional. The blood clot is then covered with HCSC, followed by a permanent restoration. Regular follow‐up is recommended. For further details on the procedure, see the ESE and AAE guidelines [37, 38].
3.3.3 Outcome
To date, the level of evidence for revitalization procedures is quite high. Besides case reports, case series, and laboratory and animal studies, retrospective and prospective cohort studies [14, 39] have been published, together with randomized controlled clinical studies [13,40–42], systematic reviews, and meta‐analyses [2, 43, 44]. Survival rates and healing are similar for the apical plug and revitalization. Apical closure can be observed after both procedures, but increased root length and thickness is seen only after revitalization. However, this finding is infrequent and unpredictable (Figure 3.2). Comparative clinical studies show higher success rates for the apical plug and revitalization compared to CH apexification [26], with similar results across the former two modalities [14, 27].
Failure with recurrent signs and symptoms of inflammation is a possible outcome after revitalization [34, 45]. Insufficient disinfection may be considered the main cause. Illustrative animal studies show that the presence of bacteria in the root canal after revitalization, which may not manifest in periapical lesions, will cause an absence of root lengthening and thickening [46].

Figure 3.2 Revitalization in a necrotic immature central incisor. (a) Preoperative radiograph. (b) Postoperative radiograph after provocation of bleeding and application of hydraulic tricalcium silicate (Biodentine, Septodont, Saint‐Maur‐des‐Fossés, France). (c) 12‐month and (d) 24‐month follow‐up. Completion of root formation cannot be observed. The tooth responds to cold testing.
Source: Paul Cooper, Henry F. Duncan, Matthias Widbiller, Kerstin M. Galler.
3.3.4 Limitations
Initial expectations for revitalization procedures were high, and true regeneration of the dentine–pulp complex with differentiation of odontoblasts from stem cells of the apical papilla and formation of tubular dentine was assumed. When the first animal studies, along with human teeth extracted after revitalization procedures, showed ectopic tissue formation in the root canal [32–34] – including fibrous tissue and apposition of cementum or ingrowth of bone – disillusion began to spread. Diogenes et al. [10] separated patient‐based, clinician‐based, and scientist‐based expected outcomes, and gave rise to a different perspective. Nowadays, the absence of signs and symptoms of inflammation, together with the healing of periapical lesions, is considered the primary goal of revitalization [38], and this can be achieved reliably in 91–94% of cases [2, 43].
Further limitations arise from the risk of discolouration, which may be induced by the different irrigants and materials used during the procedure. Stringent material selection (e.g. nondiscolouring intracanal medicaments and HCSC without bismuth oxide as radio‐opacifier) can help, and colour changes may be prevented by sealing the dentine in the access cavity with a dentine‐bonding agent [47, 48].
As revitalization is a fairly new treatment modality, long‐term results are not available, and the clinical protocol is still in a state of flux. Additional information will lead to slight changes to the recommended procedure. This is made explicit in the ESE position statement: ‘As … new evidence is still emerging, this position statement will be updated at appropriate intervals. This might lead to changes to the protocol provided here’ [37].
3.4 Material Requirements
3.4.1 Materials and Applications
The treatment of nonvital immature teeth presents a range of challenges. The disadvantageous crown‐to‐root ratio, thin root canal walls, and wide‐open apices make it technically difficult to place a root canal filling, as an apical stop against which filling materials can be condensed is absent. Therefore, the traditional apexification method (described in Section 3.2) aims to induce a mineralized tissue barrier at the root canal end through long‐term application of CH. The mechanical barrier facilitates root canal obturation and compaction of root filling materials without extrusion of materials into the periapical tissues.
CH used as an intracanal dressing is a mixture of a powder and a vehicle. The white odourless powder exhibits basic properties (∼pH 12.5) and dissociates into calcium and hydroxyl ions when in contact with aqueous fluids. Besides water‐soluble substances (water, saline, etc.), viscous (glycerin, polyethylene glycol, etc.) and oil‐based (silicone oil, olive oil, eugenol, etc.) solvents have been used as vehicles [49, 50]. The vehicle with which the CH powder is mixed determines the physical and chemical properties of the resulting paste. In general, the low viscosity of water‐soluble substances provides high and rapid ion liberation, whereas more viscous vehicles prolong this action. Viscous CH preparations can remain in the root canal for several months with a stable pH and a slow‐ion release, but their action may be compromised by negative side effects and limited antibacterial activity [49, 50]. Notably, the application of CH over a prolonged time span comes with mechanical weakening of the dentine and an increased risk of cervical root fractures in immature teeth [18, 51]. Furthermore, the required time for apical closure is unpredictable (6–24 months), and the risk of microleakage or bacterial contamination during the interappointment phase is high [49, 52]. CH formulations containing bismuth‐based radiopacifers may thus lead to tooth discolouration and should therefore be avoided [53, 54].
For these reasons, the apical plug technique using MTA has proven the method of choice in recent years. Here, MTA is placed apically at open foramina of immature teeth to create an artificial barrier that allows for prompt root canal obturation. Treatment time appears to be shorter compared with CH apexification, improving patient compliance, and the mentioned disadvantages recede [25, 55]. An immediate adhesive seal of the root canal and access cavity may prevent potential fracture of immature teeth [56]. MTA is a type 1 HCSC and is composed of Portland cement with bismuth oxide as radiopacifier, which characteristically sets in a moist environment. Its main components, tricalcium silicate and dicalcium silicate, react with water and form calcium silicate hydrate and CH during the setting reaction [57]. However, original commercial formulations of MTA exhibit considerable drawbacks, including a long setting time (up to three hours), difficult handling, high cost, and risk of tooth discolouration [58].
To overcome these drawbacks, improved formulations of HCSCs have been introduced over the last few years. Natural raw materials such as Portland cement are avoided in order to prevent contamination with trace elements or unwanted substances [58, 59]. Instead, manufacturers rely on pure, laboratory‐grade tricalcium silicate, which shows a similar hydration pattern to MTA and similarly forms CH [60]. Furthermore, the radiopaque bismuth oxide is substituted, because of the risk of tooth discolouration [58, 61]. Bismuth oxide interacts with collagen in the tooth structure [62] and blood [63], and also with sodium hypochlorite [64, 65] used as an irrigant, leading to the development of a dark brown/black precipitate that penetrates the tooth structure [62]. Zirconium oxide and tantalum oxide have been used as alternatives, but they exert a lower radiopacity. These radiopacifiers are inert during the setting reaction and do not leach out from the cements as described for bismuth oxide [58], and thus pose a lower risk of discolouration. Mixing liquids are supplemented with calcium chloride or calcium nitrite in order to accelerate setting, and addition of water‐soluble polymers improve their handling [58].
HCSC formulations are clinically available in powder–liquid‐set, preencapsulated, and other delivery systems. Recently, light‐curable cement preparations have been developed to simplify application through a quicker and defined setting. However, light‐curable products are generally resin‐based, meaning cement hydration and therefore CH release are limited by polymerization [66, 67]. Furthermore, resin‐based materials are disadvantageous in contact with vital tissue because of their resinous constituents, which may compromise cellular viability and biological activity [68, 69]. Other additives, such as calcium phosphates and microsilicia, have been used, but these can affect formation of CH and compromise biocompatibility [70]. The treatment outcome after the use of CH and MTA in immature teeth with pulp necrosis has been well documented. Although apical plug therapy provides several practical advantages over CH apexification, such as a lower risk of root fractures and a shorter treatment time, systematic reviews did not find any significant differences in terms of clinical success [55, 71].
A novel biology‐based alternative for immature teeth with pulp necrosis is revitalization. Here, bleeding into the root canal is induced and a blood clot forms, which serves as a biological scaffold to guide the ingrowth of reparative tissue. This regenerative endodontic approach can facilitate healing of periapical lesions and enable further development of the fragile tooth roots, providing biological and mechanical advantages [51, 72]. In this specific application, HCSCs are suitable for covering the intracanal blood clot before the access cavity is sealed with an adhesive composite restoration. In contrast to apical plug therapy, bioactive cements do not come in contact with vascularized and structured connective tissue, but instead with a loose fibrin‐based coagulum and blood cells. This may require different qualities of bioactive materials, both biologically and mechanically.
3.4.2 Biological Requirements
3.4.2.1 Bioactivity
Bioactivity describes the controlled interaction of materials with a tissue in order to initiate desired biological reactions, such as biomineralization, hydroxyapatite formation, antibacterial effects, or an immune response [73]. Thus, the goal of CH apexification is a stimulation of the vital periapical tissue in order to form a mineralized barrier. When CH contacts connective tissue, its high pH causes a superficial coagulation necrosis. Starting from a localized and mild inflammatory reaction, periapical cells begin to form osteocementum or bone‐like tissue [49]. Interestingly, MTA and other HCSCs form CH by hydrolysis of tri‐ and dicalcium silicate [58, 74]. Thus, the bioactivity of HCSCs can partly be tracked back to similar mechanisms to that of CH and lasts as long as their setting reaction is in progress. However, the abrupt ending of a setting reaction by light‐curable formulation is likely to compromise the bioactivity of the material [66, 68].
3.4.2.2 Reaction with Tissue Fluids
Another aspect of bioactivity is founded in the precipitation of carbonated apatite on the material surface when it comes in contact with body fluids or tissues. In vitro, similar observations have been made upon immersing materials in a serum‐like solution, the so‐called simulated body fluid (SBF). Within the setting reaction of the cements, hydrolysis and ion exchange lead to the formation of CH, which creates an alkaline environment [58, 75]. At this basic pH, an amorphous calcium silicate hydrate gel layer develops on the surfaces of calcium silicate particles and binds calcium ions from surrounding fluids [76]. However, the bound Ca2+ from the set cements can be released again at a later stage and reacts with HPO42− from phosphate‐containing liquids. The resulting amorphous calcium phosphates, which are in solution at first, densify near the cement surface and finally deposit on the calcium silicate hydrate layer. Over time, the nucleated calcium phosphate undergoes maturation into carbonated apatite [76]. Since carbonated apatite represents the biological elements found in bone, cementum, and dentine, it plays a triggering role in cytocompatibility and the bioactive potential of HCSCs [75, 77]. In vitro, SBF corresponds to human blood or plasma, which is generally saturated with calcium and phosphate ions [76]. Following the implantation of a foreign material into the body, the material’s surface is immediately coated with proteins derived from blood and interstitial fluids. In contrast to a bioinert material, which is encapsulated by fibrous tissue, bioactive materials create an interface that interacts with the host tissue biologically and mechanically [76–78]. Although the in vitro bioactivity of HCSCs has been demonstrated, data on the bioactive potential of HCSCs in vivo are sparse; however, recent studies indicate that the clinical situation is different between the two scenarios [76, 79, 80].
3.4.2.3 Release of Dentine Matrix Proteins
Another mechanism by which biological responses can be elicited is the release of bioactive molecules from the dentine matrix. HCSCs create an intimate interface with dentine and can solubilize signalling molecules from it by local demineralization [81, 82]. Dentine is a rich source of cytokines, bioactive proteins, and growth factors that have been fossilized during dentinogenesis [83, 84]. Once released, these can exert their biological effects on various cell types and promote, for example, differentiation of pulp cells into an odontoblast‐like phenotype [85–87]. Like MTA, soluble components of setting and set HCSCs have a basic pH and are able to release calcium ions, which lead in turn to the release of dentine matrix components (DMCs) [88, 89]. These are likely to have an impact on reparative and regenerative processes during the treatment of immature teeth.
3.4.2.4 Blood Clot
HCSCs can be applied in various treatment strategies and environments. Not only can they be placed as an apical plug at the root tip, in direct contact with bone or connective tissue, but they can also be used to cover a blood clot at the CEJ during revitalization therapy. Thus, local conditions, as well as neighbouring tissues, may alter the material setting and formation of carbonated apatite. There is a paucity of literature, however, on the behaviour of HCSCs in contact with blood or autoresorbable collagen plugs, which are usually placed on a clot to create an abutment for cement application. In this context, the status of Portland cement in teeth that had been extracted for orthodontic purposes after revitalization therapy was assessed chemically and micromechanically, showing a porous cement surface that was primarily enriched in calcium carbonate instead of CH or apatite [80]. Interestingly, very little attention has been paid to calcium carbonate products, and their role in bioactivity is yet to be determined [90]. Moreover, blood contamination can affect the antimicrobial properties of HCSCs, which may be clinically relevant [91].
Further studies are required to elucidate the behaviour of these materials in the context of revitalization, but it appears both desirable and necessary to develop materials with optimal properties for this specific purpose.
3.4.3 Mechanical Requirements
3.4.3.1 Impact on Microhardness
Although the setting reaction of calcium silicate cements is hydraulic and requires a wet environment, unwanted liquid contamination at the place of application can affect the mechanical characteristics of the restorative material. Indeed, failure of the setting reaction and reduction of microhardness have been described in the presence of serum or tissue fluids – the very environment that the material used as an apical plug is in contact with [92, 93]. Likewise, blood components that are unavoidable in revitalization therapy can compromise mechanical properties of HCSCs [58]. Contamination of MTA with blood has been shown to deteriorate compressive strength and microhardness [94, 95].
Besides mechanical properties, adhesion of HCSCs to dentine is also important. For both the apical plug and revitalization, a sufficient bond to the dentinal surface is crucial in order to stabilize immature teeth and prevent leakage and contamination [72, 96]. HCSCs interact with dentine through mineral exchange at the tooth–material interface and the formation of mineral tags along the dental tubules [97]. The interfacial gap is filled by calcium phosphate deposition [98]. Furthermore, alkaline hydration products denature collagenous proteins at the dentine interface, as well as in tubular dentine. The exact mechanism by which the mineral interaction occurs requires further investigation, since certain laboratory‐related conditions may affect the conditions at the interface [98, 99].
In this context, newer HCSCs have demonstrated stronger bonds to dentine than conventional MTA [100, 101]. A better uniformity and a smaller size of the particles enable deeper material penetration, which generally results in cohesive failures – whereas adhesive modes prevail with MTA [100]. Interestingly, the irrigation solution applied before application of HCSCs can compromise the bond to dentine. Notably, when using MTA, chlorhexidine and EDTA were reported to affect the micromechanical adhesion; however, saline and sodium hypochlorite (NaOCl) allowed for a sufficient bond strength [100, 102, 103]. Similarly, intracanal dressing with CH increased bond strength, whilst antibiotic medicaments reduced it significantly [101]. Ultrasonic agitation during placement of HCSCs achieved better marginal adaption, which led to stronger adhesion [104].
Long‐term contact of CH pastes with dentine alters the facture resistance of the tooth. Similarly, prolonged contact of HCSCs with mineralized dentine has adverse effects by affecting the collagenous matrix [105], resulting in reduced flexural strength [106].
Handling requirements (including fluidity and setting time) and environmental issues (such as wetness, existing fluids, and dentinal anatomy) are important factors affecting the specific clinical application of HCSCs. Therefore, the development of new and customized cements will be helpful in overcoming the current drawbacks and improving clinical outcomes. In addition to a demand‐driven material design, treatment parameters such as irrigation, intracanal dressing, and application modality need to be considered and adapted. Thus, the use of CH as a medicament and a final irrigation with saline or NaOCl seem to be beneficial for an optimal dentine adhesion.
3.4.3.2 Discolouration
From a clinical perspective, discolouration of the tooth crown constitutes a major drawback that highly compromises the aesthetic result – and thus the outcome – of a procedure from the patient’s perspective. Therefore, a decisive requirement of materials used for apical plug therapy and revitalization treatment is that they avoid staining the dentine and instead preserve the natural tooth shade. The discolouration potential of HCSCs varies widely. In this context, a multitude of factors have an impact, including material constituents, contamination with blood, and mixture with irrigants, as well as local environmental factors. As already stated, bismuth oxide serves as a radiopaque additive in the original MTA formulation. It can cause tooth discolouration on reaction with collagenous dentine components, irrespective of its concentration [62, 81]. Despite other factors, newer formulations of HCSC avoid bismuth oxide and thus successfully obviate dentine staining [58, 81]. Furthermore, contamination of MTA with blood leads to inclusion of blood components in the porous structure and darkening of the tooth. However, newly developed materials reportedly have fewer pores and thus a reduced risk of discolouration when they come in contact with blood [107]. Likewise, residues of NaOCl in dentine tubules can effect colour changes, although the exact chemical nature of these changes is still unclear [61, 64, 81]. Generally, light and oxygen affect bismuth compounds and lead to the formation of a dark‐brown precipitate that can discolour the tooth [108, 109]. Interestingly, formulations without bismuth oxide have also been reported to discolour dentine when irrigated with NaOCl or CHX, but to a much lesser extent [61, 81].
These bioactive cements are placed primarily as plug materials within the root canal, but they can reach the aesthetic zone – especially in revitalization procedures, where the plug may reside at the level of the CEJ. In order to counteract discolouration, bismuth oxide should be avoided in material formulations, irrigant residues should be carefully removed before cement application, and blood contamination should be obviated. Further, the coronal dentine surface may be sealed with bonding agents [47, 48], and cements covered with opaque resin‐based composite materials.
3.5 Healing Process and Cellular Responses
3.5.1 Biological Aspects
‘Primary dentinogenesis’ is the term used to describe the process of developmental dentine formation. The odontoblasts at the periphery of the pulp reportedly deposit the dentine at a relatively rapid rate of ∼4 μm/day until tooth root formation is complete. The odontoblasts remain intimately associated with the dentine via their processes, which reside in tubules for the lifetime of the tooth. The pulp remains vital following tooth eruption and functions to support the tooth throughout its life. At the pulp core are fibroblasts, which are the most abundant cell type; these secrete the relatively soft spongy tissue matrix. Also within the pulp are complex and intricate structures of nerves and blood vessels that enter the tooth via the roots. These are more finely branched in the peripheral areas, where they intimately interact with the tissues and cells to provide the tooth with sensitivity and the nutrient supply to ensure its vitality. There are also several stem cell populations, which reportedly reside within the pulp and not only contribute to any repair and regenerative processes within it but also are capable of differentiating into other tissue cell types [110, 111].
The odontoblasts at the periphery of the tissue continue to lay down secondary dentine throughout the life of the tooth. This process reportedly occurs at a much slower rate of ∼0.4 μm/day. Importantly, cells within the viable pulpal tissue have been shown to be able to detect and respond to infecting bacteria, pain stimuli, and temperature changes. Indeed, many of these irritations can lead to the stimulation of the formation of tertiary dentinogenesis, which represents the tooth’s natural wound‐healing response [112, 113]. Two forms are described (see Figure 3.3). Following relatively mild dental injury, such as in the early stages of dental caries, the original odontoblasts reactivate and secrete a reactionary dentine at an increased rate of deposition. This newly formed dentine exhibits tubular continuity with the original primary and secondary dentines. If the dental injury is of a greater extent and intensity – such as due to a rapidly progressing and deep carious lesion – then the original odontoblasts will die beneath the site of injury. However, if the disease and tissue damage can be arrested, potentially following clinical intervention, then stem/progenitor cells from within the pulp may be recruited to the site of the tissue damage, where they are signalled to differentiate into new odontoblast‐like cells. These cells also deposit a tertiary reparative dentine matrix at a relatively rapid rate, seen clinically as a dentine or mineralized bridge which partially repairs the tooth’s hard tissue and aims to ‘wall off’ any further invading bacteria. Quite clearly, these two tertiary dentinogenic processes exhibit different degrees of complexity (Figure 3.3). Reactionary dentinogenesis more simply requires only the upregulation of the activity of the surviving odontoblasts, whilst the reparative dentinogenic response comprises complex interactions between several cellular processes, including recruitment, differentiation, and activation of dentine synthetic and secretory activity [114, 115]. Notably, the reported upregulated rate of tertiary dentine deposition is ∼4 μm/day – similar to that described for the primary dentinogenesis process [116].

Figure 3.3 Schematic of the processes of tertiary dentine formation. Reactionary and reparative dentinogenesis processes differ in the source of the secreting cell. Reactionary dentinogenesis is a comparatively simple process undertaken by the existing primary odontoblasts when a relatively mild stimulation causes their upregulation of synthetic and secretory activity. Reparative dentine formation involves a more complex sequence of events in which a severe stimulus results in the death of the primary odontoblasts, which are subsequently replaced following differentiation of progenitor or stem cells into odontoblast‐like cells under the influence of bioactive molecules (including dentine matrix components). (A) Enamel. (B) Dentine. (C) Pulp.
Decades of study have demonstrated many aspects of similarity between primary and tertiary dentinogenesis. Both processes are signalled and driven by a similar array of bioactive molecules, many of which become sequestrated and bound within the dentine during its formation [84, 116, 117]. Notably, these bioactive molecules can be released from their inactive form by a variety of treatments to the dentine, including by carious bacterial acids and certain restorative materials such as CH and HCSCs [88, 89, 118]. When released, they stimulate the formation of a dentine or mineralized bridge beneath the lesion or restored area via a reparative dentinogenic mechanism.
Studies show not only that stem cells from the pulpal niche can be stimulated by these bioactive molecules but also that gradients of many of these molecules result in their ‘trafficking’ to repair sites, as well as stimulating increases in their numbers to enable repopulation of the wounded area. Furthermore, it is clear that many of these bioactive molecules can stimulate the differentiation of the ‘homed’ stem cells into odontoblast‐like cells and promote the upregulation of their dentine synthetic and secretory activity [1]. Notably, recent studies have also indicated that when degraded due to the relatively aggressive environment generated in the wound area, these bioactive molecules – including members of several growth factor families – are more potent in terms of signalling compared to their intact counterparts [119].
Neuropeptides and neurotrophic factors are expressed by odontoblasts, potentially due to their close association with the tooth’s neuronal network (plexus of Raschkow) and to odontoblasts originating from cranial neural crest cells [120–122]. Indeed, several neuropeptides have been shown to play a role in pulpal disease pathogenesis. Studies have examined the presence and functionality of a range of these molecules within the dentine–pulp complex, including calcitonin (CT), calcitonin gene‐related peptide (CGRP), neuropeptide Y (NPY), substance P (SP), and vasoactive intestinal polypeptide (VIP) [123]. Interestingly, some have been shown to be capable of stimulating regenerative dentinogenic processes. SP, CGRP, VIP, and NPY may represent novel regulators of pulpal angiogenesis, whilst CGRP and CT have been shown to stimulate osteodentine deposition [124, 125]. Furthermore, targeted studies on the neurotrophins of nerve growth factor (NGF) and brain‐derived neurotrophic factor (BDNF) have demonstrated their expression in dental pulp cells and odontoblasts during tooth development [122]. NGF has been implicated in dentinogenesis and dental repair, and studies suggest that it may directly promote odontoblast differentiation [126–128]. Glial cell line‐derived growth factor (GDNF
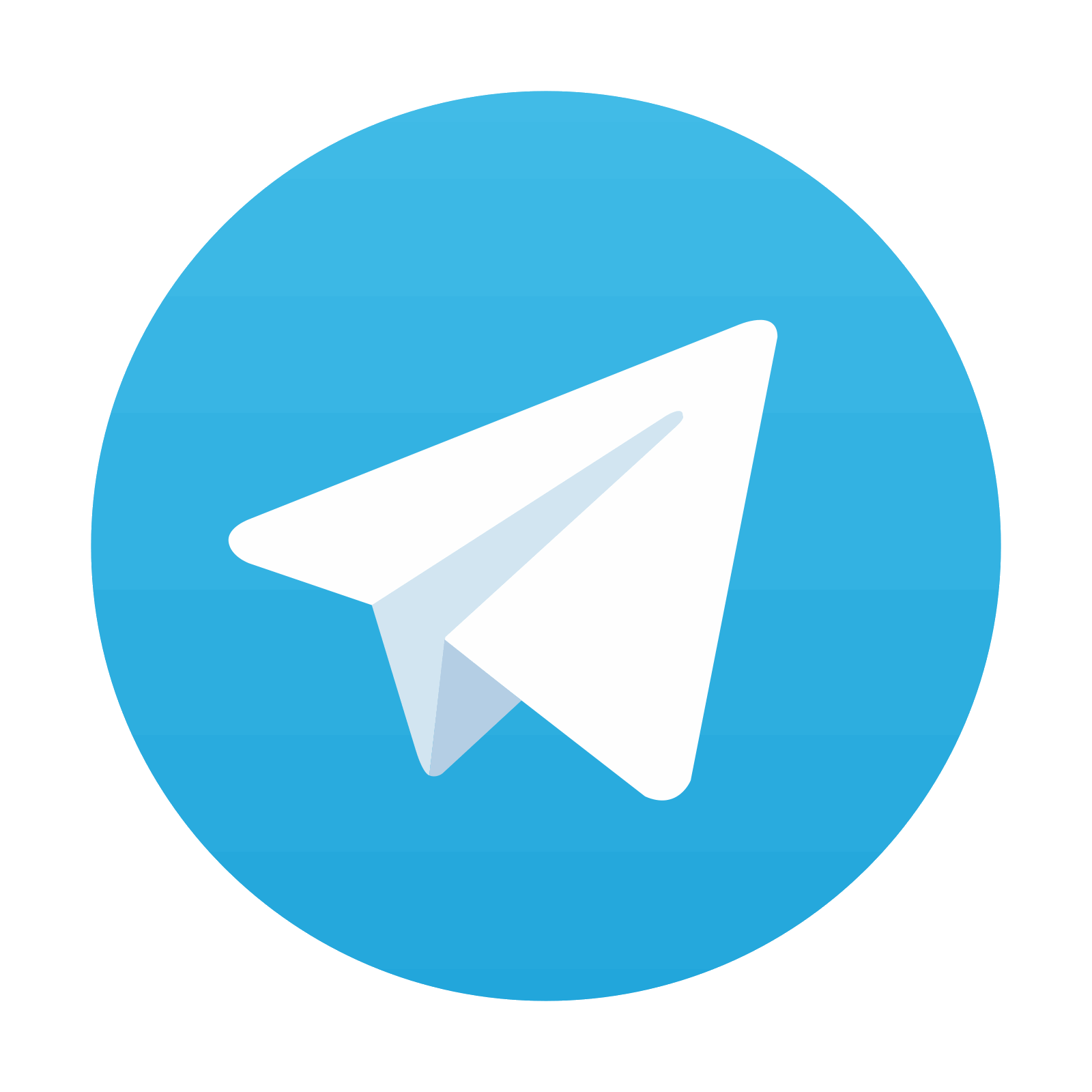
Stay updated, free dental videos. Join our Telegram channel

VIDEdental - Online dental courses
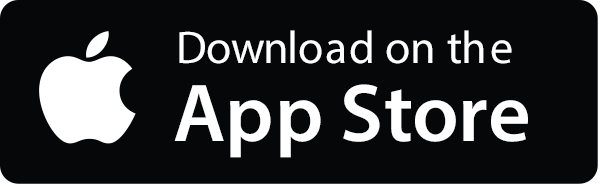
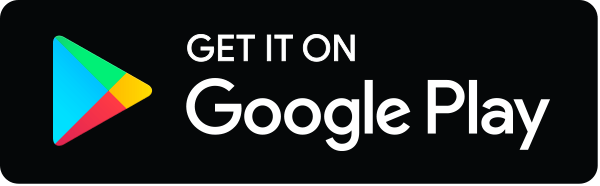