Introduction
In this study, using a cone-beam computed tomography system, we evaluated the airways of 30 adults.
Methods
The shapes of the 3-dimensional volume of the airway were analyzed and compared among the subjects by using surface superimposition software techniques.
Results
The airway had the greatest variability in the hypopharynx, in the region below the epiglottis, and above the vocal folds. Moderate variation was apparent at the nares, behind the soft palate, and at the base of the tongue. Conservation of form was seen at the central portion of the nasal airway surrounding the inferior turbinate.
Conclusions
The potential for comparing the shape of the airway among subjects is possible.
Many studies in orthodontics have focused on the airway and its potential importance to modifying the development of the craniofacial region. Much of this research has studied how the airway is implicated in developing abnormally long vertical facial dimensions. Orthodontists have also been actively involved in evaluating the airway and treating subjects with particular types of sleep apnea. With the advent of low-radiation, rapid computed tomography (CT) scanning, the potential for orthodontists to assess craniofacial growth in 3 dimensions is now available, and with that analysis is the capability of evaluating the complete airway.
Determining the airway from images generated by cone-beam CT (CBCT) includes defining the most effective anatomic landmarks. Many previous studies have evaluated the airway by using 2-dimensional cephalometric analyses. Tourné showed that the bony nasopharynx extends from hormion (the most dorsal contact point of the vomer on the body of the sphenoid) to the level of the hard palate and foramen magnum. The pharynx’s structural volume increases by approximately 80% during growth. Transverse pharyngeal growth at the pterygoid hamulus is completed at 2 years of age. Choanal width increases about 23% by maturity with remodeling at the pterygoid laminae. The sagittal diameter of the nasopharynx, measured from the posterior nasal spine to basion, increases only 9% because of the influence of cranial base flexure (angle formed by sella to nasion to basion), since an acute angle leads to more vertical pharyngeal development. Hormion is displaced dorsally, increasing the length of the roof of the nasal cavity, but the posterior nasal spine has an even greater dorsal displacement. The vomer’s dorsal border and the clivus become upright, and the concurrent descent of the posterior nasal spine and basion with age tends to reduce the angle of the nasopharyngeal roof, but this might be cancelled with bone apposition at the pharyngeal aspect of the clivus. This growth counters the contribution of the growth of the spheno-occipital synchondrosis to nasopharyngeal depth, and probably contributes to most of the increase in pharyngeal depth. The vertebral body of the atlas might better represent the true posterior limit of the anteroposterior dimension of the pharynx, but the growth effects are similar. The contribution of apposition at the posterior palatine border is minimized relative to growth at the transpalatal suture and the tuberosities. This backward growth effect is compensated by secondary maxillary anterior displacement from cranial base growth. The main growth direction of the pharynx is vertical, with downward movement of the palate and primarily vertical growth at the spheno-occipital synchondrosis. Growth at these areas increases the height of the bony nasopharynx by 38% during childhood, and this dimension accounts for most of the increase in pharyngeal capacity, continuing until 13 years of age (female) or 18 years of age (male).
Adenoid vegetation is the most frequently cited cause of airway obstruction. The lymphoid tissue increases rapidly in size during infancy, slows thereafter, peaks before adolescence, and declines in the adult. Some evidence from cephalometric studies suggests that the adenoids increase during the preschool and primary grade years and then involute during preadolescence and adolescence. Lymphoid tissue does facilitate velopharyngeal closure but can result in blockage of the airway. The absolute size of the adenoids is not as important as the relative space that the mass occupies in the nasopharynx. Adenoid obstruction appears to be most common among children with a long face and small nasopharynx. Persons with the long-face syndrome have smaller nasopharyngeal cavities than those with a square face, so that even a small increase in adenoid bulk could easily cause airway obstruction.
When the airway is threatened to a sufficient degree or at another level than the oropharynx, the postural adaptation of tongue and hyoid will fail to cope, with purely nasal respiration becoming an oronasal breathing pattern, with compensations including parting of the lips, a drop in the mandibular rest position, lower or more forward tongue position, and extension of the head and neck.
Measurement of nasal obstruction has included evaluating cross-sectional area, peak nasal flow, nasal resistance, and respiratory mode (identifies nasal/oral airflow ratio). Direct measurement of the ratio of oral to nasal airflow is an objective measure of respiratory function.
Warren et al constructed a model of the upper airway with a variable nasopharyngeal isthmus and a variable oral port to determine the effects of airway size and shape on the aerodynamics of simulated breathing, and to develop a theoretical basis for predicting when breathing mode will change from nasal to predominantly oral. Several theoretical predictions resulted from their model. A nasal airway cross-sectional area less than 0.4 cm 2 might represent an inadequate airway in adults, and mouth breathing would be expected. The amount of adenoid obstruction must be great to affect airway resistance. If airway resistance in the nose were high, large adenoids would be a serious airway problem and induce mouth breathing. When nasal airway resistance is high (≥4.5 cm H 2 O/L/s), the mouth will open about 0.4 to 0.6 cm 2 , shifting a significant amount of air orally and reducing airway resistance to a normal level. If morphologic changes are caused by airway impairment, other factors such as a large tongue, large tonsils, or a long, draping velum, could be significant contributing factors.
These studies can now be complemented by 3-dimensional (3D) evaluation of the airway in a subject seeking orthodontic treatment. Many techniques are effective in evaluating the airway volume and include cine-magnetic resonance imaging, magnetic resonance imaging, spiral CT, and optical coherence tomography. In this article, we propose 1 method using CBCT to compare the 3D airway among subjects.
Material and methods
CBCT data sets acquired from 30 patients were used in this study. Some scans of patients with pathologic conditions were included, with 4 being evaluated for temporomandibular disorders, 1 with a pneumocoele of the right maxillary antrum, and 1 with an odontogenic cyst surrounding an impacted mandibular third molar. Scans of subjects with developmental conditions such as impacted teeth and orofacial clefts (1 subject) were permitted. Selection to homogenize the sample with respect to age, sex, and ethnicity was not specifically performed. The sample included 13 male and 17 female subjects with an average age of 22 years 8 months ± 12 years 1 month (median, 19 years 2 months). Most were white (17), followed by Asian (6), Hispanic (5), and African American (2). The most common reasons for referral for the CBCT scan were impacted teeth (10 subjects) and temporomandibular joint or temporomandibular disorder evaluation (8 subjects).
CBCT data was acquired from 2 systems: the NewTom QR DVT 9000 (Aperio, Sarasota, Fla) in a private radiology laboratory in Sacramento, California, and the CB MercuRay (Hitachi Medico Technology, Tokyo, Japan) at the Division of Orthodontics, University of California at San Francisco. The patient is supine in the NewTom and upright with the CB MercuRay; this could modify the shape of the airway. However, the primary purpose of this study was to demonstrate the variability in airway shape and the methods to compare these shapes.
The entire airway as a single unit was threshold-segmented by using the CT numbers of each voxel and edited by hand with each transverse slice to remove any visible extraneous scatter, artifacts, or background, similar to the method described by Meehan et al. For areas with widely different thresholds, a combination of threshold segmentation and manual slice editing was used to obtain refined surfaces with minimal artifacts. The segmentation process involved using the sculpt tool of CBWorks (CBWorks 1.0; CyberMed Inc., Seoul, Korea) to narrow the scan into a volume of interest including only the structure that was to be segmented. Threshold segmentation tools were used to isolate the osseous structures and the airway space from the data. Details in this approach have previously been published. After segmentation, the resulting set of masks (highlighted areas representing each structure of interest in each image slice of the CBCT scan) was rendered into a shaded surface mesh in the CBWorks’ Shaded Surface Display tool. The 30 resulting surface meshes were exported from CBWorks in a Virtual Reality Modeling Language (.vrl) format into Amira software (version 3.1, Mercury Computer Systems, Berlin, Germany) for further analysis.
Due to limitations in the ability of the software to solve the problem of comparing nonhomeomorphic surfaces (surfaces that do not correspond in topology and cannot be transformed into one another by continuous and invertible mapping) or numbers of nodes (each voxel comprising the surface becomes a node in a tetrahedral mesh in which each voxel is connected to its 3 nearest neighbors), a reference structure was selected for indirect comparison of the individual surfaces. The reference surface consisted of 1 surface model of 1 patient, who was considered average as determined from a lateral view. The reference surface was nonrigidly transformed into each individual surface to provide a set of 30 surfaces of identical topology with the same number of nodes. The nonrigid transformation was completed by embedding 27 landmarks in each airway by using the Amira landmark tool ( Table ). Landmarks were embedded pairwise in the arbitrary reference and in each individual structure. The Amira landmarksurfacewarp tool was set in a rigid transformation method to align the surfaces based on the embedded landmarks. Individual surfaces were then enlarged or compressed uniformly in the x-, y-, and z- dimensions by hand with the Amira transform editor dialog to scale each surface to the same size as the arbitrary reference surface.
Landmark | Description | Bilateral point |
---|---|---|
NarAM | Anteromedial vertex of the impression of the naris | Yes |
NarPM | Posteromedial vertex of the impression of the naris | Yes |
NarL | Lateral vertex of the impression of the naris | Yes |
NasA | Most medioinferior point on the reflex of the anterior nasal airway | Yes |
ConPS | Posterosuperior aspect of impression of inferior nasal concha | Yes |
ConPI | Posteroinferior aspect of impression of inferior nasal concha | Yes |
IAM-S | Most superior point on impression of internal auditory meatus | Yes |
IAM-I | Most inferior point on impression of internal auditory meatus | Yes |
FR-S | Most superior point on the base of the impression of Rosenmüller’s fossa | Yes |
FR-I | Most inferior point on the base of the impression of Rosenmüller’s fossa | Yes |
FR-D | Most dorsosuperior point at the height of the impression of Rosenmüller’s fossa | Yes |
PW-C | Greatest convexity on the impression of the lateral aspect of the posterior pharyngeal wall | Yes |
PA | Most lateroinferior point at the junction of the impressions of the soft palate and anterior pharyngeal wall | Yes |
BT | Most lateroinferior aspect of the impression of the base of the tongue | Yes |
EJ-L | Most lateroinferior point on the impression of the esophageal junction | Yes |
EJ-C | Most inferior point at the central aspect of the impression of the esophageal junction | No |
VF-L | Most posterolateral point on impression of vocal folds | Yes |
VF-A | Most anteroventral point on impression of vocal folds | Yes |
Ad | Most dorsosuperior point on impression of adenoid tonsil | No |
Once the surfaces were aligned and scaled, a subset of 7 landmarks was used to identify the mathematically “most average” surface and to rank the remaining surfaces relative to it. Because the objects were now of similar alignment and size, the remaining differences in the surfaces were primarily related to differences in shape. To simplify the analytic calculations, these 7 landmarks, representing the basic form of each structure, were defined and used with all 30 forms including 4 shapes that did not extend fully rostrally and caudally. The x, y, and z coordinates of the 7 landmarks (Ad, left and right NasA, ConPS, ConPI, IAM-S, PA, and BT; Table ) and the rectangular coordinate values were used.
By averaging the coordinate data for each data set, the centroid of each coordinate set was determined ( Fig 1 ). Mean centroid coordinates were determined by averaging the coordinate values of the individual centroids. All data sets were centered at a common centroid located at the origin by subtracting the individual centroid coordinates from each landmark’s coordinates. The distance for each landmark of each set to its corresponding centroid was then calculated. The average distance of all landmark points in each set to the centroid was determined to be the centroid size of the data set. If scaling was correct, the data sets should be of identical centroid size. Manual scaling was generally within 3% to 5% of the calculated mean centroid size. Therefore, a correction factor (ratio of the mean centroid size to the individual centroid size) was applied to the coordinate values of each data set to scale all sets perfectly to a common centroid size. These steps of aligning the individual coordinate systems about a common centroid and scaling them to a common centroid size constituted a Procrustes transformation of the coordinate data.
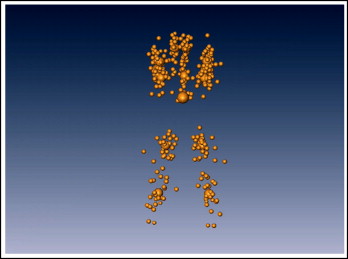
The root mean square error of each data set relative to the set of average landmark coordinates was determined. Root mean square error provides a way to determine how well the mean coordinate values fit each data set and provides part of the information necessary to rank the surfaces. Because root mean square error is always positively signed, arranging data sets from least to greatest root mean square errors orders each set in increasing distance from the mean but does not indicate whether each set is smaller or larger than the mean or average landmark coordinates ( Fig 2 ). To give a sign to each root mean square rank, the average x, y, and z coordinates for each landmark were subtracted from each landmark coordinate. The signs of the difference for each component of each coordinate were recorded as more than 0 (assigned a value of 1) or less than 0 (assigned a value of −1). The sign of the sum of the landmark x, y, and z component differences was set to be the sign of the root mean square error. The signed root mean square errors were then ranked from 1 to 30 ( Fig 3 ). The data set with the smallest root mean square error was the reference surface for the surface-warping procedure.
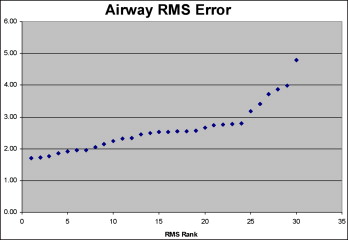
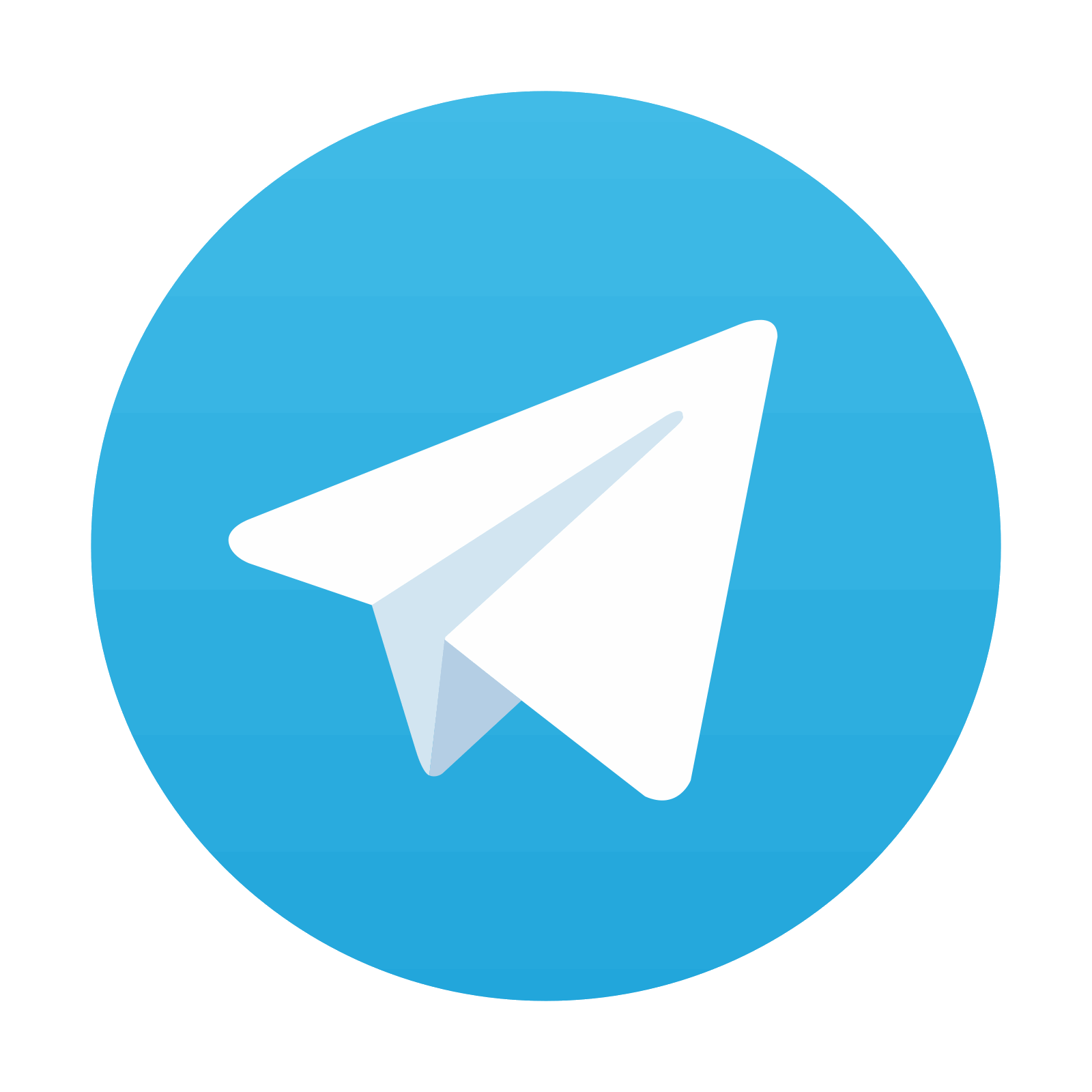
Stay updated, free dental videos. Join our Telegram channel

VIDEdental - Online dental courses
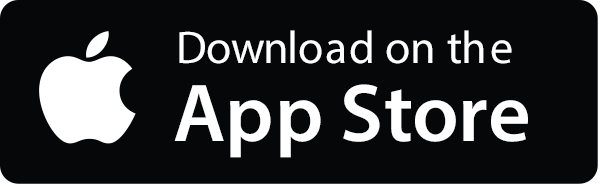
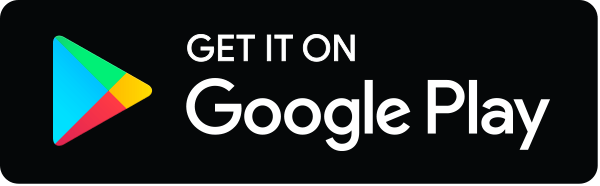
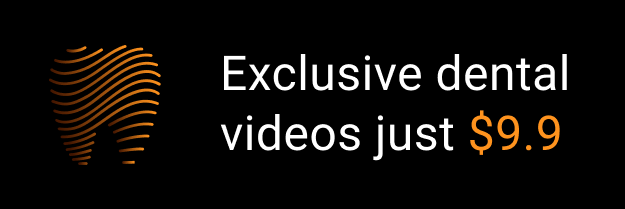