Fig. 7.1
Proposed self-healing concept containing nano-encapsulated healing agent. (a) Crack formed due to damage, (b) nano-capsule is ruptured releasing healing agent that migrates to crack, and (c) healing agent is then polymerised after contacting a catalyst (Reprinted from Samadzadeh et al. (2010). With permission from Elsevier)
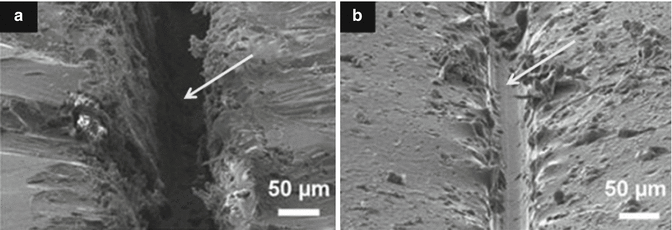
Fig. 7.2
SEM micrographs of micro-crack healing. (a) Coating containing scratch defect (arrowed) and (b) self-healed scratch defect after healing process (Reprinted from Samadzadeh et al. (2010). With permission from Elsevier)
Interpenetrating polymer networks incorporating linear polymers (e.g. poly(methacrylated phenyl glycidyl ether)) has shown diffusion of the linear polymer phase into the crack interface as a form of self-healing process. SEM images (Fig. 7.2) of damaged areas clearly demonstrate that healing of micro-cracks in the order of 20 μm can be achieved, from mobile chain interdiffusion and entanglement (Peterson et al. 2012).
Polymers incorporating embedded microcapsules of liquid monomers are also used to induce self-healing properties. When the material is damaged, the microcapsules release monomers into the damaged areas and subsequently repair the damage and prevent further fracture. Other approaches include the “mechanochemical” activation of a catalyst in a polymer chain, i.e. when mechanically induced chain scission occurs, a catalytic site is activated, thereby facilitating polymerisation of acrylic monomers. Other approaches are supra-molecular (i.e. non-covalent interactions) and also methods to exploit covalent bond forming repair processes (Colquhoun and Klumperman 2013).
Both intrinsic and extrinsic mechanisms can be used to develop self-healing properties. Polymer architecture such as chain stiffness and cross-link functionality, cross-linking density and content or reversible groups and multiphase polymer structure all have an influence on the self-healing ability of various polymer systems (Garcia 2014). Other approaches incorporating shape memory materials, swollen materials or passivation can also be utilised to impart self-healing properties, and a possible mechanism for GIC repair is shown in Fig. 7.3. The investigation of poly(ethylene-co-methacrylic acid)-based ionomers has also indicated its ability to self-heal (Wu et al. 2008). However, it is yet to be seen how manufacturers will incorporate these new technologies into GICs in an effort to improve their mechanical and adhesive performance.
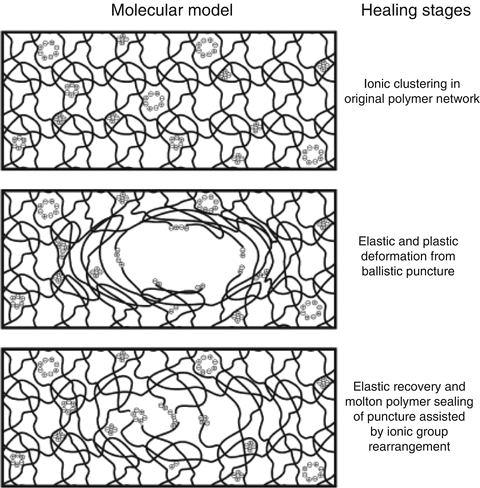
Fig. 7.3
Theoretical mechanism of self-healing in glass-ionomer materials (Reprinted from Wu et al. (2008). With permission from Elsevier)
7.10 Other Novel Polymer Networks for Improvements in Strength and Other Properties
In order to increase the physical properties of GICs, improvements can be achieved by modifying polymer chemistry contained in the liquid component or in the solid form present in the powder component (Guggenberger et al. 1998). Multi-arm poly(acrylic acid-co-itaconic) acids have been prepared and have demonstrated improvements in compressive strength for conventional cured GICs (Xie et al. 2010), and star-shaped poly(carboxylic acid) polymers have been proposed for resin-modified GICs (Weng et al. 2014). Vinyl-containing poly(acrylic acid-co-itaconic acid) copolymers have also shown higher flexural strength leading to a tougher fracture surface and plastic deformation properties (Wu et al. 2003). The polymer chemistry here is complex (Yelamanchili and Darvell 2008), and future improvements in results rely on the interactions between the multicomponent nature of different GIC systems which contain many components, including complex initiating systems.
7.11 Porosity Reduction
As glass-ionomers are mixed together in order for the acid–base reaction to proceed, typically, small voids or air pockets are incorporated into the mix. Although the effects of these voids are not exactly understood, it is assumed that these have a negative effect on the GIC strengths. One method of reducing these voids is to provide both parts of a glass-ionomer in a paste version, which can be hand-mixed by a spatula or through some form of a static mixer (Boehm et al. 2011). However, compared to encapsulated delivery systems, this form of delivery is typically uneconomical and is not widely used. A major advancement for encapsulated versions would be the reduction of voids during the extrusion of the mixed paste, although no such capsule version has yet to be developed.
7.12 Improvements in Fracture Toughness
Fracture toughness is known to be an important material property for successful posterior load-bearing restorations (Lloyd and Adamson 1987). To date, it has been questioned whether the survival of GICs will reach levels achieved by composite resin materials in load-bearing situations (Burke 2013). However, in some studies modern GICs used to restore load-bearing cavities have been demonstrated to perform satisfactorily up to 10 years. This demonstrates that wear properties simulated in a laboratory do not necessarily translate to reduction of anatomical form or surface roughness in real-life situations (Burke 2014).
For primary molar restorations, recent studies have indicated that the survival rate for amalgam restorations is no different from that of atraumatic restorative treatment with a high viscosity (HV) GIC. For both amalgam and HVGIC, the main reason for failure is mechanical, and it has been suggested that for single surfaces of primary teeth, HVGIC is a viable alternative to amalgam (Yamazaki et al. 2006).
Modifications to the polymer component of the GIC have shown to improve the fracture toughness of GICs. Acrylic acid-itaconic acid-N-vinylcaprolactam (NVC) ter-polymers have been synthesised and have shown significantly higher plane-strain fracture toughness (KIc) at 1-day and 1-week storage time periods compared to the control (Fuji IX, GC Corp, Tokyo, Japan) (Moshaverinia et al. 2010). However, issues associated with viscosity increases need to be overcome for the incorporation of any of these new polymers.
Improvements of fracture toughness depend on many variables. It has been shown that modification of the polymer components, such as amino acid-containing GICs, methacryloyl amino acid reactive diluents and a series of other new chemistries are viable paths to improving properties such as fracture toughness of the final cement (Culbertson 2001).
Other novel polymer systems such as 6-arm star-shaped poly(acrylic acid) polymers have also shown significant increases in fracture toughness compared to commercial RMGICs, similar in value to composite material (Zhao et al. 2009). Adjustment of the molecular weight (MW) of polymers has also been investigated for improving properties, although it is unknown how these changes will affect clinical outcomes.
Viscoelastic (i.e. viscous and elastic response of materials to deformation) properties of GICs have also been reported in an effort to understand the mechanical behaviour of these materials. Fracture may involve wear and adhesive de-bonding modes of failure, as well as the typical bulk fracture of the various GIC materials; both GIC and RMGIC exhibited viscoelastic properties (Yamazaki et al. 2006). Modifications in the powder, for example, particle size reductions and powder to liquid ratios, have also been shown to increase fracture toughness in GIC formulations (Mitsuhashi et al. 2003).
Interfacial fracture toughness has also been studied in order to provide paths for GIC improvements related to failure by fracture. Formulations are investigated at adhesive interfaces, and typically the fracture then continues into the bulk material (Setien et al. 2005; Cheetham et al. 2014b). These studies are extremely relevant for development of improved GIC materials. To improve the longevity of GICs, properties such as fracture toughness of the bulk material and the adhesive properties (interfacial fracture toughness or interfacial work of fracture) need to be improved.
7.13 Improvements in Adhesion to Dentine and Enamel
The critical issue with adhesive dentistry is to place the adhesive material using a method that gives the best chance for long-term stability and the creation of an “effective seal” to the adhesive–tooth interface. In the case of resin-based adhesive systems, there are several strategies that have been proposed in order to optimise the probability of success, and these include application of MMP inhibitors and collagen cross-linkers, additional enamel etching, agitation of the adhesive for deeper penetration, etc. (Manuja et al. 2012). These approaches are also very relevant when trying to improve the bonding reliability of GIC materials. Many original and review articles are available to explain the different concepts involved in adhesion to dentine and enamel (Tyas 2003; Manuja et al. 2012; Atmeh et al. 2012).
Adhesion to dentine and enamel is a complex science and similar to adhesively bonded composite dentistry; successful outcomes depend on many variables (Perdigão 2010; De Munck et al. 2012). Glass-ionomers have also been shown to give a more durable bond compared to other adhesive systems (Van Meerbeek et al. 2010). However, much is unknown about this bond and most importantly the degradation mechanisms of the bond.
The bonding mechanism of new-generation GICs has now demonstrated resin tag formation. Studies investigating the differences between dentine conditioned with phosphoric or polyacrylic acid solutions have shown that both protocols create a surface that allows RMGIC resin tags (Fig. 7.4) to form (Hamama et al. 2014; Korkmaz et al. 2010; El-Askary and Nassif 2011). Other studies demonstrate the intimate chemical interaction with dentine and enamel surfaces (Fig. 7.5) with exposure of collagen due to dentine pretreatment, with the remaining hydroxyapatite being used as “receptors” for chemical bonding of the GIC. Micropores have also been shown to increase the ability for micro-mechanical bonding (Van Meerbeek et al. 2003).
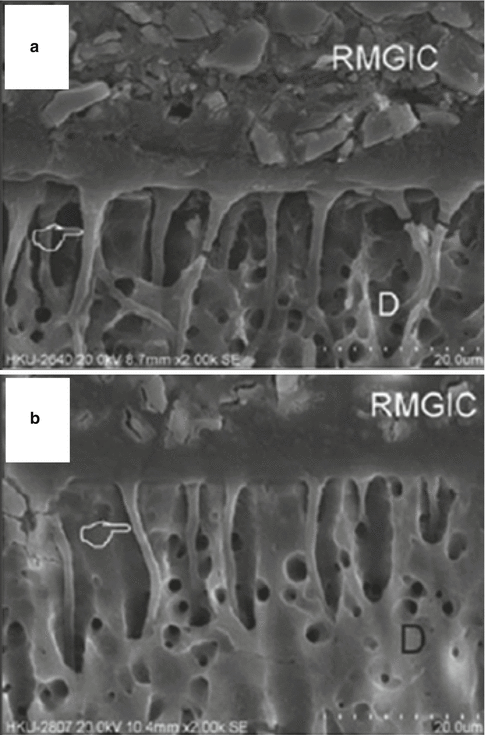
Fig. 7.4
SEM micrographs showing the interface between RMGIC and dentine. The top image (a) had dentine treated with 37 % phosphoric acid, the bottom image (b) was treated with a solution of 25–30 % polyacrylic acid conditioner (D denotes dentine). The hand pointer indicates the presence of resin tags (Reprinted from Hamama et al. (2014). With permission from John Wiley & Sons)
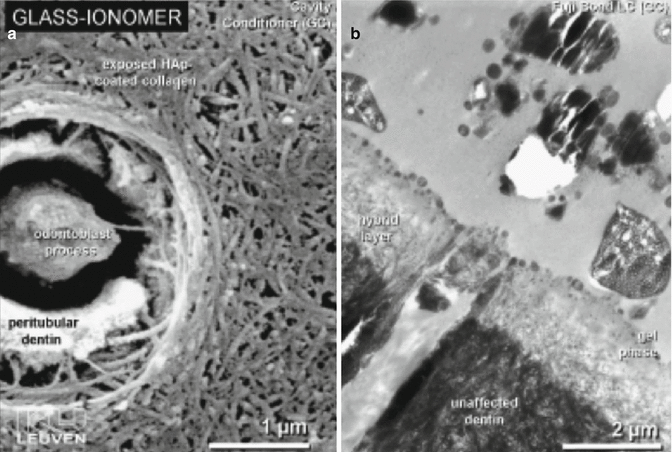
Fig. 7.5
(a) SEM image showing the effect of polyacrylic acid conditioner on dentine and (b) TEM photomicrograph showing the hybrid layer and gel phase created after RMGIC placement (Reprinted from van Meerbeek et al. (2003). With permission from Operative Dentisitry, Inc.)
The chemical interaction and formation of ionic bonds between carboxylic acid groups with hydroxyapatite have been described previously, and future GICs will use this information to provide materials with stronger, more resilient bonding interfaces (Yoshida et al. 2000).
Further improvements of the bonding to hydroxyapatite, enamel and dentine components will continue to be focused on the incorporation of new polymer chemistries into the GIC formulations. Pioneering work investigating the binding energies of functional groups to these structures as well as the molecular structure of the polyalkenoic acid component has shown that these factors significantly affect the chemical bonding to hydroxyapatite structures (Fukuda et al. 2003; Sennou et al. 1999). This process to gather information on surface interaction will continue to be used to modify resin formulations and optimise chemical adhesion processes for GICs.
Apart from chemically related improvements, other “macro” physical observations of GICs may be utilised to achieve improvements in adhesion. Spherical bodies (Figs. 7.6 and 7.7) have also been shown to be involved in GIC adhesive surfaces which could provide fracture initiation sites for bond failure, although their participation in the adhesive process is not yet fully understood (Yiu et al. 2004a, b). New materials will try to eliminate these porous, randomly incorporated features which disrupt a relatively homogenous and compact solid material. Further understanding of the interfacial properties of GICs with enamel and dentine will inevitably lead to improving the longevity of the GIC bond.
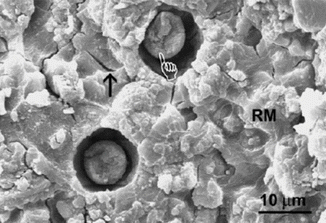
Fig. 7.6
SEM micrograph showing a RMGIC surface fractured adjacent to the GIC–dentine interface, showing the presence of spherical bodies (hand pointer). Dehydration cracks are identified with an arrow (Reprinted from Yiu et al. (2004a). With permission from Elsevier)
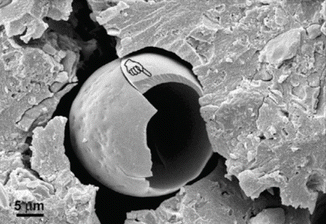
Fig. 7.7
SEM image of a spherical body showing brittle fracture of the spherical structure. The handpointer identifies a fractured eggshell-like structure within the GIC at the GIC–dentine interface [Reprinted from Yiu et al. (2004b). With permission from Sage Publications]
Research on GIC adhesion provides information for understanding the fundamental mechanics and kinetics of the bond formation and subsequent path of bond degradation in order for new adhesive strategies to be devised. Short-term, static bond strengths give limited information when comparing the potential longevity of a GIC bond and understate its adhesive abilities. A fracture mechanics approach, including interfacial fracture toughness and work of fracture testing (Fig. 7.8) showing the “total energy” required to initiate fracture, provides more relevant information on the possible improvements of the bond durability (Cheetham et al. 2014b).
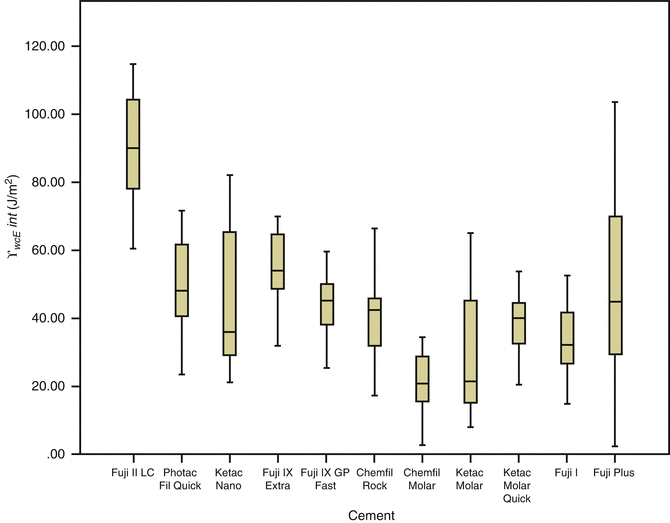
Fig. 7.8
Comparison of the interfacial work of fracture (γ wofint) results (J/m2) together with standard deviations for glass-ionomer bonded to dentine (Reprinted from Cheetham et al. (2014b). With permission from Elsevier)
7.14 Future Delivery Systems
The delivery format of glass-ionomers varies from powder–liquid bottle versions and single-use encapsulated systems to dual-barrel syringes (and unit-dose) for paste–paste GICs. Paste–paste systems generally reduce the porosity of the final GIC paste, and single-use formats (Fig. 7.9) have been recently commercialised (Boehm et al. 2011). However, these formats have not been as widely accepted as single-use encapsulated delivery formats for powder–liquid versions of GICs.
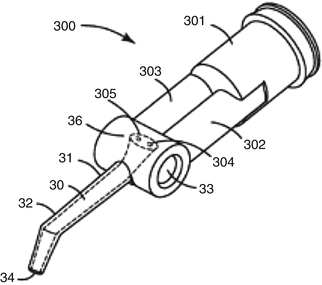
Fig. 7.9
Example of a single-use dual-barrel paste–paste delivery system (Reprinted from Boehm et al. (2011))
Encapsulated systems eliminate the need for measurements to be made by the user, resulting in a dispensed material with more reproducible features such as setting reaction (gel and set time), strength and paste consistency. The other benefits of current GIC capsule systems are the ease of direct delivery and their single use. New-generation GIC capsules (Fig. 7.10) will be able to mix “ultra” high viscosity GICs, which could also allow for a new GIC with very high powder to liquid ratios and strengths not seen in GICs before (Cheetham 2014).
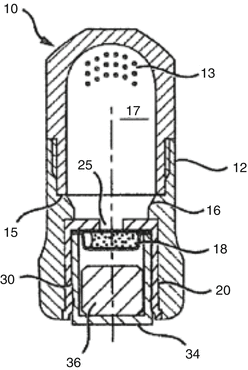
Fig. 7.10
Example of a single-use powder–liquid delivery system (Reprinted from Cheetham (2014))
However, a disadvantage of “capsules” is that during the mixing process, they typically introduce some form of subclinical micro-porosity in the paste. Various methods, such as centrifuging the capsule after the mixing process, or vacuum mixers, have been employed to reduce this with limited effect (Suzuki et al. 2004). Theoretically, the reduction of this porosity will increase the physical properties of GICs. The clinician is yet to see the direction of future designs of GIC delivery systems, but it is likely that ease of the use in terms of delivery and reduction of porosity are areas where improvements can be made.
7.15 Wear Improvements
Currently, it is popular to place a coating over the setting GIC in order to protect it during the initial setting stage, which improves the immediate aesthetics of the restoration and acts as a method to fill in any porosity created on the surface (Zoergiebel and Ilie 2013). However, the effect of this coating on GICs placed on occlusal surfaces has not always been found to be significant when comparing the wear of uncoated surfaces (Diem et al. 2014). Enamel–GIC margins may also be protected with resin coatings, although this adds an extra step in the placement of GICs (Hokii et al. 2014).
Recent laboratory tests (Fig. 7.11) have demonstrated that the current commonly used commercial GIC materials continue to have inferior wear resistance to amalgam. However, in two-body wear studies, the wear resistance of some GICs is now similar to compomers, with recommendations that these GICs may be used adequately in occlusal restorations of primary teeth (Lazaridou et al. 2015). Furthermore, it was found that GIC materials covered with a filled polymer did not perform better in terms of GIC vertical loss (μm) and volume loss (mm3). The mean vertical loss of the best performing GIC was still nearly three times worse than amalgam, and when comparing volume loss, the amalgam had six times less volume loss (Lazaridou et al. 2015). Hence, commercial GIC materials remain inferior in wear situations when compared to amalgam. What is interesting from these studies is that when compared to other posterior materials, some GICs can now be viewed as an alternative option.
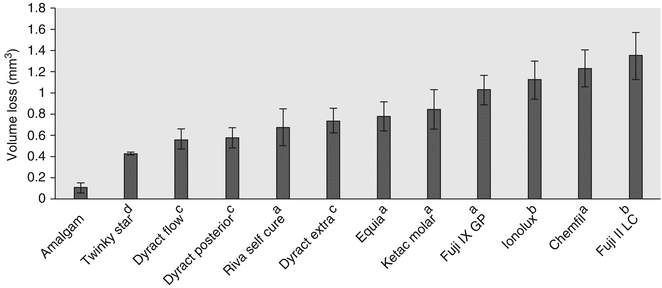
Fig. 7.11
Mean volume loss (mm3) and standard deviations of dental materials after an oral simulated wear test. Note superscript a refers to conventional GIC, b refers to RMGIC, and c refers to polyacid-modified resin composite material (compomer) and d resin composite material (Reprinted from Lazaridou et al. (2015). With permission from Springer Science)
For some time, manufacturers and researchers have been attempting to improve the wear characteristics of GIC materials. Significant advances have been made since initial tests demonstrated that GICs were brittle and showed catastrophic failure during wear events, concluding that they were not acceptable for posterior occlusal applications (McKinney et al. 1987). Future efforts to improve the difference between amalgam and GIC wear properties will continue to be focused on by researchers. Wear can be improved by increasing the hardness of the GIC surface prior to any wear event. This can be achieved in a number of ways, and increasing the powder to liquid ratio, concentration of polyacids or the molecular weight of polyacids have been reported as possible ways (Guggenberger et al. 1998; Smith 1998). As commercial materials contain undisclosed proprietary compounds and are produced in novel processes, much of these advances are not documented in the public domain.
Silver particles have been utilised to provide a means of lubrication during wear events, and commercial products containing silver alloys have been shown to provide lower wear resistance (McKinney et al. 1988; Xie et al. 2000). However, from an aesthetic perspective, the addition of metal particles compromises the clinical outcome, and these materials are not currently widely accepted.
Studies by Xie et al. in 2000 have also shown that the more integrated the GIC microstructure is, the higher the mechanical properties. Furthermore, large glass particle systems have been described as contributing to lower wear properties. The integrity of the interface between the glass filler system and the polymer, the particle sizes of each of the filler systems and the number of voids created during mixing can all influence the wear properties of current GICs (Xie et al. 2000). As GICs age, their wear rate decreases, and differences in wear rates have been described in terms of polyalkenoic acid content, the “overall” chemical composition of the GIC and differences in the filler system and size of fillers used (van Duinen et al. 2005).
Avenues for future improvements in the wear properties of GICs will focus on innovations in these areas described above, in order to provide a harder external surface of GIC restorations, although it is unlikely that the wear properties in the short term will reach those obtained by modern amalgam alternatives.
7.16 Aesthetic Improvements
Compared to modern composite resin materials, the aesthetics of GICs are typically inferior, and much work is being performed in order for GICs to produce aesthetic restorations that blend in with tooth structure. For the future of GICs, the aesthetic properties are an important feature as patients in the “post amalgam age” are demanding that their teeth are restored with more tooth-like materials.
Aesthetics with GICs is likely to continue to evolve. For example, the colour change over time may be reduced with the addition of new polymer and surface chemistries that allow GICs to resist colour change even when subjected to staining media such as beverages or certain foods.
The initial translucency and optical properties (Fig. 7.12) of RMGICs are now similar to leading composite resin materials (Ogledzki et al. 2012). The optical properties, such as translucency and opacity values, together with closer colour matching of shades to Vita™ shades may be improved to follow composite resins. Conventional-cured GICs still lack the translucency properties (Fig. 7.13) of modern-day composites, although improvements are being introduced.
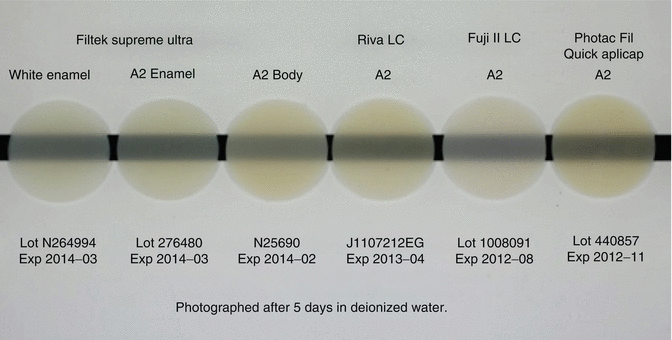
Fig. 7.12
Opacity comparison of a commercial composite resin (Filtek Supreme Ultra) and RMGICs (Riva LCTM, Fuji II LCTM, Photac Fil Quick AplicapTM) indicating current RMGIC materials can achieve similar opacity levels compared to resin composite (Image courtesy of SDI Limited R&D)
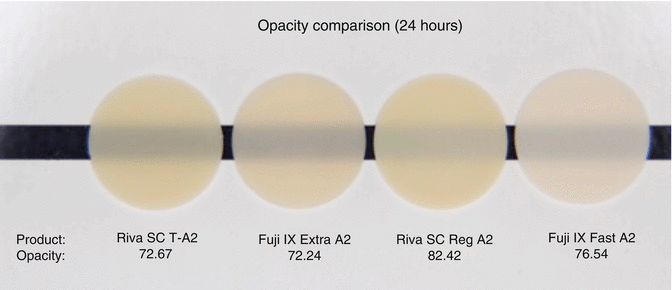
Fig. 7.13
Opacity comparison of commercial GIC demonstrating that they have higher opacities compared to RMGICs shown in Fig. 7.12. All materials are conventional cured type (Image courtesy of SDI Limited R&D)
7.17 Fluoride Release
It is well documented that GIC materials release fluoride sourced from their glass system or from additional additives such as sodium fluoride in the set cements (Williams et al. 2002; Jones et al. 2003; Guida et al. 2002). Recent 1-year fluoride release data (Fig. 7.14) of commercial GICs has shown significant differences in fluoride release between current commercially available GICs (Shiozawa et al. 2013). Furthermore, the release of Sr, Na, Al and Si ions has been shown to affect the properties of the glass-ionomers and demonstrate how the GIC can interact with its aqueous environment. This ionic transfer is a unique feature of glass-ionomers not seen in composite resin materials. Although most manufacturers highlight the fluoride release of their material, future glass-ionomers could be designed to release high levels of calcium, phosphate or other ionic species considered beneficial to certain cavity conditions (Forsten 1998).
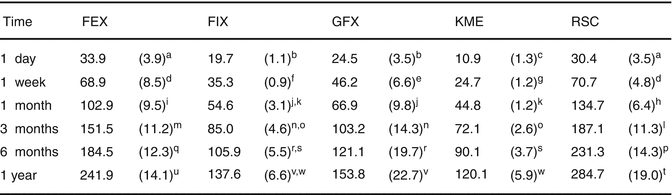
Fig. 7.14
Cumulative fluoride release (μg/cm2) of various commercial GIC materials up to 1 year. Standard deviations are in parentheses, and the same superscript letter denotes homogenous subsets (p > 0.05) (FEX: Fuji IX GP EXTRA, GC; FIX: Fuji IX GP, GC; GFX: GlasIonomer FX-II, Shofu; KME: Ketac Molar Easymix, 3MESPE; RSC: Riva Self Cure, SDI) (Reprinted from Shiozawa et al. (2013). With permission from Springer Science)
The fluoride release from GICs has been shown to decrease with time, which in turn decreases their antimicrobial effectiveness (Dionysopoulos et al. 2013). To counteract this, it has been demonstrated that GICs can “recharge” their fluoride release by topical fluoride application (e.g. fluoride-containing toothpaste) (Dionysopoulos et al. 2013; Arbabzadeh-Zavareh et al. 2012). Studies have shown that covering aged GIC with 0.1 % fluoride toothpaste or 1.25 % fluoride gel significantly increases the fluoride release (Seppa et al. 1993).
Glass-ionomer cements based on strontium glass systems have also been shown to release strontium ions originating from the glass structure to the adjacent tooth structure. Although these ions do not have any antibacterial effect, it is envisaged that they are rapidly exchanged for calcium ions, and a synergistic relationship with fluoride could facilitate antimicrobial properties (Dabsie et al. 2009). New-generation GICs will aim to maintain a constant relevant supply of fluoride ions over their life, without degradation of the cement, and any improvement in the release of fluoride should be incorporated into future GICs.
Addition of potassium and fluoride has also been previously made to the liquid components of GICs, and this has demonstrated ion release up to 500 days (Williams et al. 1999). Glass-ionomers immersed into potassium fluoride exhibited ion release twenty times greater than specimens that had additions to their liquid component, and this process could be adapted for pre-reacted GIC filler systems that act as reservoirs for specific ions (Williams et al. 1999).
7.18 Bioremineralisation/Biopromoting Improvements
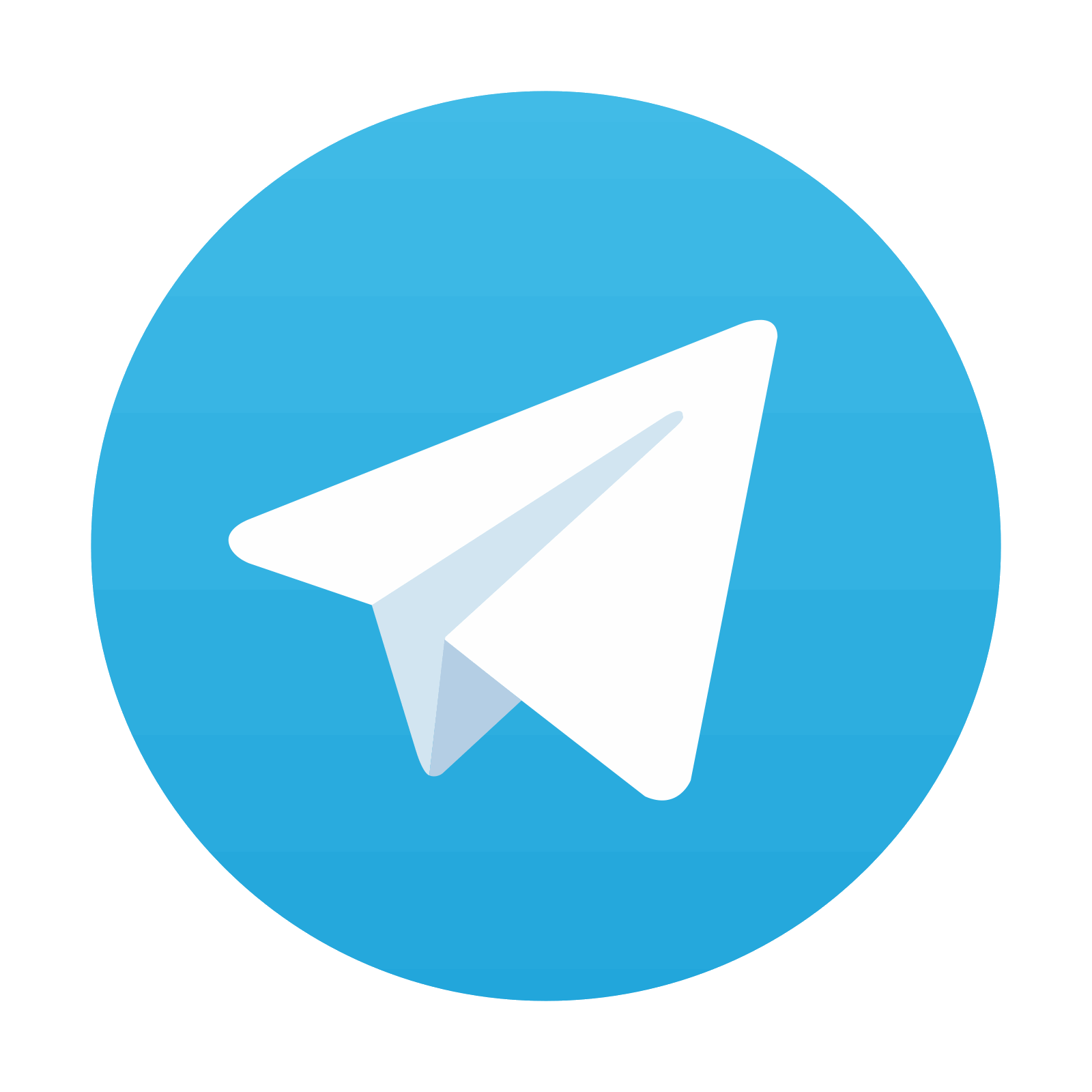
Stay updated, free dental videos. Join our Telegram channel

VIDEdental - Online dental courses
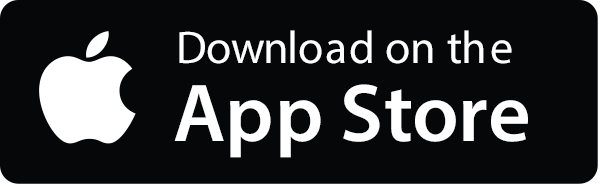
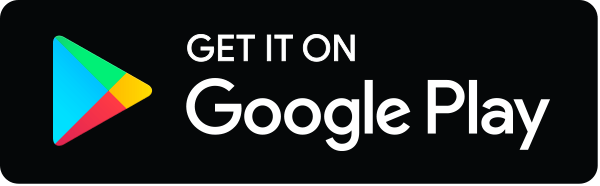