Revascularization and Healing of Orthognathic Surgical Procedures
- Donald J. Hull
- Raymond J. Fonseca
Orthognathic surgical procedures were first popularized in the European literature in the early 1920s but were not commonly performed in the United States until the 1960s. Along with a rise in the popularity of these procedures came a variety of empirically based techniques in an effort to improve outcomes and minimize complications. The work of Kole, and later Bell and colleagues, provided the scientific basis for interpreting the clinical observations of these procedures and the foundation for their further modification. The dramatic development of orthognathic surgery since the 1970s can be attributed to the scientific understanding of its biologic basis.
Our discussion of the biology of orthognathic surgical wound healing begins first with a basic review of wound healing and proceeds by describing how the restoration of tissue integrity and functional capacity has been seen specifically after orthognathic surgery.
WOUND HEALING
At its most basic level, the healing of injured tissue is achieved by one of two processes: regeneration or repair. Regeneration is achieved when the healed tissue is structurally and functionally indistinguishable from the original tissue. In contrast, repair describes the process of reestablishing tissue integrity through the formation of a fibrous connective tissue scar.
PHASES
Although wound healing involves myriad intricate biochemical processes, it has classically been described in terms of three overlapping, interdependent phases: inflammatory, proliferative, and remodeling ( Figure 2-1 ).
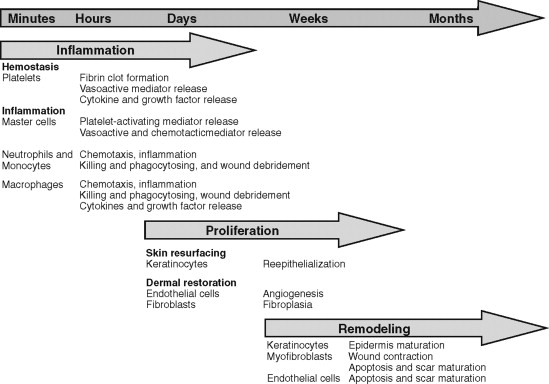
The inflammatory, or initial, phase begins immediately upon tissue injury. Under normal circumstances this phase lasts 3 to 5 days but in some cases may persist for weeks. The inflammatory phase can be further subdivided into vascular and cellular phases.
The primary vascular response to tissue injury and bleeding is vasoconstriction and the initiation of hemostasis. In addition to slowing blood flow to the area through vasoconstriction, injury to vessels initiates hemostasis through fibrin clot formation, which is followed by coagulation. Fibrillar collagen, fibronectin, and other adhesive proteins in the extracellular matrix of the damaged vascular wall are exposed to and activate platelets. The activated platelets undergo adhesion and aggregation as well as release (1) other mediators that stimulate further aggregation (e.g., adenosine diphosphate, thromboxane A 2 ) and activate the coagulation pathway (e.g., fibrinogen, von Willebrand factor VIII) as well as (2) cytokines that play an important role in later phases of wound healing, including platelet-derived growth factor and transforming growth factor–alpha (TGF-α). Bleeding is stopped by a fibrin clot formed through the coagulation cascade’s conversion of soluble fibrinogen to insoluble fibrin. Vasoconstriction, which is mediated by a multitude of reactants such as serotonin, histamine, and prostaglandins, lasts 5 to 10 minutes and is quickly followed by vasodilation. This dilation allows the influx of leukocytes and other mediators that help create open spaces between endothelial cells, resulting in plasma and leukocyte migration into the interstitial tissues. Together these induce the classic signs of inflammation: erythema (rubor) from the dilation of blood vessels, warmth (calor) from the increased blood flow, swelling (tumor) from the transudation of fluid, and pain (dolor) from the release of bradykinin and histamine as well as the pressure created by edema. This ushers in the cellular phase of inflammation.
The cellular aspect of the inflammatory phase is characterized by the invasion of leukocytes into the area of injury. This influx involves both polymorphonuclear and mononuclear leukocytes but is initially predominated by polymorphonuclear leukocytes, more specifically neutrophils. Later, the monocytes that accompany the neutrophils are transformed to macrophages and become the predominate cell after 2 to 3 days.
The neutrophils and monocytes are attracted to the site of injury by chemotactic factors released during hemostasis. These cells marginate , or cling to the sides of the blood vessels, and then migrate into the extracellular space by the process of diapedesis . Once in the interstitial tissue the neutrophils degranulate, releasing lysosomal enzymes and proteases to destroy bacteria and foreign bodies. As the process continues, macrophages phagocytose foreign organisms, tissue debris, and remaining neutrophils. Macrophages are considered the most important regulatory cell in the inflammatory process because in addition to this phagocytosis, they release a host of chemotactic and growth factors that attract fibroblasts and induce the formation of granulation tissue and angiogenesis. In doing so, macrophages begin the transition to the proliferative phase of healing.
The proliferative, or intermediate, phase of healing involves reinforcement of the wound, reestablishment of blood supply, and reepithelialization. Reinforcement of the wound occurs by fibroplasia, the process of migration of the fibrin clot, mesenchymal cell differentiation to fibroblasts, and synthesis of structural proteins, mostly extracellular matrix and immature collagen. This process is mediated by a number of cytokines, including platelet-derived growth factor, TGF-α, TGF-β, and tumor necrosis factor.
The lattice created in fibroplasia provides support of the reestablishment of blood supply, or angiogenesis. Angiogenesis occurs by the budding of endothelial cells of existing vessels at the periphery of the wound. Among other things, this process is stimulated by hypoxia and cytokines such as vascular endothelial growth factor. The formation of vascular anastomoses helps deliver nutrients to further fuel fibroplasia. The result is a cellular, edematous, and highly vascular tissue commonly referred to as granulation tissue. The collagen of granulation tissue initially is deposited in a haphazard manner lacking structure and strength. As a result, an overabundance of collagen is laid down to increase wound strength. The rate of collagen synthesis that began at 3 to 5 days after wounding peaks at approximately 21 days and then rapidly declines. Although the rate of collagen production peaks, as a balance between synthesis and destruction by collagenase is reached to begin the remodeling phase the tensile strength of the wound is only approximately 15% of the original tissue at 3 weeks. Thus the remodeling phase is important for the recovery of wound integrity.
Although the remodeling phase is referred to as the terminal phase of healing, remodeling begins almost immediately after injury. Remodeling describes the process of changing the content and integrity of the healing tissue over time. The platelet plug is broken down and replaced by a fibrin clot that is lysed as it is ingrown by vessels and collagen to form granulation tissue, which is transformed into scar by wound contracture and abundant collagen production. The revision of this scar tissue over months and years is described as the remodeling phase. During this process the overabundant type III collagen is replaced by type I collagen, and cross-linking between collagen fibers increases, leading to a decrease in scar bulk and an increase in strength. The tensile strength of a wound is approximately 40% of the original tissue at 1 month but can reach its plateau strength of 70-80% of uninjured tissue at 6 months to 1 year ( Figure 2-2 ).

CATEGORIZATION
From a clinical point of view, wound healing can be categorized as occurring by first, second, or third intention. First intention, or primary healing, occurs when a wound created by a sharp instrument has its edges approximated to near-anatomic position within hours of injury. Under these circumstances minimal epithelialization, collagen deposition, contraction, and remodeling are necessary for healing. Surgical incisions closed by suture and accurately reduced bone fractures held with rigid fixation are examples of healing by primary intention.
Healing by secondary intention is accomplished when a gap is left between wound edges or tissue loss occurs that prevents approximation of the wound edges. This healing requires filling the defect with new connective tissue, requiring epithelial migration, collagen deposition, contraction, and remodeling. The healing of extraction sockets and non-reduced bony fractures are examples of healing by second intention.
Healing by third intention often involves avulsive or contaminated wounds that are left open to begin healing by secondary intention and are later revised to close and heal by first intention. This process of healing by tertiary intention also is known as delayed primary closure.
BONE HEALING
Bone is one of the few tissues in the body that, under the proper conditions, heals by regeneration rather than repair. Two type of healing can occur in bone fracture or osteotomy: indirect and direct. Osseous healing after orthognathic surgery often involves both.
Indirect bone healing occurs between displaced bony segments with no intersegmental contact. The space is filled with a hematoma that clots and provides a matrix for infiltration of inflammatory and mesenchymal cells much like that described in soft tissue healing. The segments are splinted by a soft tissue callus composed of fibrous tissue and cartilage. The callus undergoes extensive remodeling in its ultimate conversion to lamellar bone. During this process osteoclasts, which are multinucleate giant cells originating from monocytes, resorb the ends of the segments while the fibrous callus is converted to more rigid fibrocartilage. The fibrocartilage is gradually replaced as osteoblasts differentiate from adjacent pluripotent mesenchymal cells and lay down osteoid. The mineralization of osteoid results in immature woven bone that is eventually converted to organized lamellar bone through Haversian remodelling. Indirect bone healing is prevalent in most maxillary osteotomies.
Direct bone healing does not involve callus formation and requires reapproximation and rigid fixation of the bony segments. Even in cases of intended perfect anatomic reduction, such as with fractures, areas of direct bone contact and small areas of intersegmental gaps occur. Contact healing occurs between the points of direct intersegmental contact without cellular or vascular ingrowth. Lamellar bone is directly formed by Haversian remodeling between the segments. Gap healing involves ingrowth of vessels and mesenchymal cells without osteoclastic activity. Osteoblasts quickly begin depositing osteoid, which forms woven bone in larger gaps but can directly form lamellar bone in smaller gaps. Direct bone healing occurs between well-adapted proximal and distal segments in mandibular sagittal split osteotomies.
As with soft tissue healing, the complex process of degradation and synthesis seen in bone healing is driven by a host of growth factors, cytokines, and mechanical stimulation. Any minute movement between segments was thought to impaired bone healing. And although high tensile strength does induces fibrous tissue production, low levels of tensile strain have been shown to promote bone formation. Low-level tissue strain may be necessary for cell differentiation and callus remodeling.
MAXILLARY SURGERY
HISTORY OF MAXILLARY OSTEOTOMIES
In 1859 a procedure was described for the removal of nasopharyngeal polyps. Although von Langenbeck did not realized it, he had performed the first orthognathic surgical procedure. David Cheever followed suit in 1867 with a maxillary osteotomy for treatment of complete nasal obstruction. These procedures were used for the treatment of neoplastic pathologic conditions. The use of maxillary osteotomies for the correction of dentofacial deformities would not occur until the twentieth century.
In 1921 Cohn-Stock introduced the anterior maxillary osteotomy. Now the focus was obtaining a functional occlusion. This segmental approach also was advocated by other surgeons. The posterior maxillary osteotomy was used to correct dento-facial deformities but was not used until 1959 by Schuchardt.
Wassmund introduced his Le Fort I, or total maxillary osteotomy technique, in 1927. Axhausen used a similar technique in 1934 to correct a healed maxillary fracture. He reported complete mobilization with immediate repositioning. However, maxillary orthognathic surgery was not widely practiced. Segmental osteotomies, either anterior or posterior, were used instead of the total maxillary osteotomy, largely because of the fear of bone necrosis and devitalization of teeth. Many surgeons believed that the maxilla healed by fibrous union. Complete mobilization was avoided to prevent a compromise in blood flow. Unfortunately, these early procedures had a high incidence of relapse, probably because of tension from a lack of sufficient mobilization.
In 1965 Obwegeser suggested complete mobilization of the maxilla so that repositioning could be accomplished without tension. This proved to be a major advance in stability as documented by de Haller, Hogemann and Willmar, and Perko.
Maxillary procedures performed in the 1960s used a pedicle of soft tissue to preserve the blood supply to the osseous segments. Bell’s research from 1969 to 1975 helped establish the biologic basis for orthognathic surgery. Before Bell’s research, procedures were largely based on clinical experience. Adult rhesus monkeys were studied at various postoperative intervals. Both histologic and microangiographic data were collected to study the wound healing process. The data showed early osseous union and small areas of transient intraosseous ischemia that had resolved by 2 weeks after surgery. These studies demonstrated that as long as the maxilla is pedicled to the palatal mucosa and the labial gingiva and mucosa, complete downfracture and mobilization of the maxilla can be accomplished with adequate vascular supply. Furthermore, stretching the vascular pedicle, transecting the incisive canal, and transecting the descending palatine vessels have no discernible effect on revascularization or bone healing. Armed with this knowledge, surgeons could now perform the total maxillary osteotomy with less concern.
ANATOMIC CONSIDERATIONS: VASCULAR STRUCTURES
The direct blood supply of the structures of the maxilla stem from the branches of the external carotid artery ( Figure 2-3 ). The facial and ascending pharyngeal arteries come off the external carotid below the level of the maxilla. The ascending palatine artery, a proximal branch of the facial artery, anastomoses with the ascending pharyngeal as they ascend to anastomose with the lesser palatine artery after it emerges from its canal in the posterior palate.
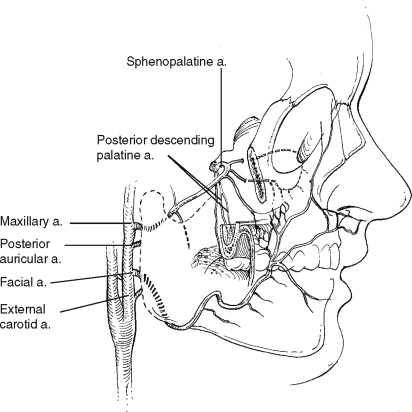
As the external carotid artery ascends, it gives off the internal maxillary artery. This vessel traverses the infratemporal fossa and passes through the pterygomaxillary fissure into the pterygopalatine fossa, where it branches into its terminal vessels. With rare exception the terminal branching occurs in the following order: the posterior superior alveolar artery and the infraorbital artery travel anteriorly, the descending palatine artery travels inferiorly, and the sphenopalatine and vidian artery travel medially. The sphenopalatine artery gives off the nasopalatine artery, which travels inferiorly through the incisive canal in the anterior maxilla ( Figure 2-4 ).
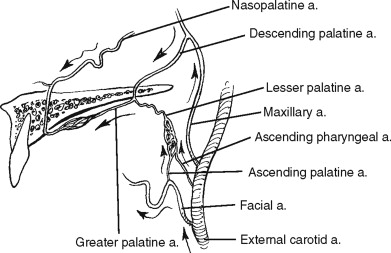
LE FORT I OSTEOTOMY
REVASCULARIZATION AND HEALING
Vascular perfusion of injured tissues is a critical factor in healing and has been of paramount concern since the earliest attempts at orthognathic surgery. Thus careful incision design and surgical technique are critical in maintaining vascular anastomoses between hard and soft tissues. Complications from vascular ischemia after Le Fort I osteotomy include delayed healing, loss of gingival tissue, periodontal defect, loss of teeth, and loss of bony segments.
The most extensive studies evaluating revascularization and healing of the osteotomized maxilla were done in the early 1970s. Bell and colleagues demonstrated that early osseous union with minimal transient osteonecrosis occurred after total maxillary osteotomy, indicating that the palatal soft tissue pedicle and the labial buccal gingiva provide an adequate nutrient pedicle for single-stage osteotomy.
Further studies showed that sufficient blood supply was maintained after stretching of the palatal soft tissue pedicle, segmentalization of the maxilla, and bilateral transection of the palatine artery even though these factors were thought to induce aseptic necrosis. The microangiographic evidence that bilateral transaction of the descending palatine vessels did not adversely affect Le Fort I osteotomy provided by Bell et al in 1975 was further demonstrated by Quejada et al in 1986, You et al in 1991, and Bays in 1993. Dodson et al measured blood flow to the maxillary gingiva by laser Doppler flowmetry during and after Le Fort I osteotomy with sacrifice of bilateral descending palatine arteries. Their results were similar to those of previous investigators, showing transient ischemia and restored blood flow in the anterior maxilla 1 week postoperatively.
In 1995 Bell et al continued to investigate the limits of the Le Fort I osteotomy technique by using standard circumvestibular incision, segmentalizing the maxilla, stretching the vesicular pedicle, and transecting the descending palatine arteries. The result was uncomplicated postoperative healing with only transient ischemia. The vascularity of the maxillary segment was again studied by Siebert et al in 1997. These perfusion studies were intended to elucidate the palatal contributions to the blood supply of the mobilized Le Fort I maxillary segment. They found the vascular contributions to be the ascending palatine branch of the facial artery and the anterior branch of the ascending pharyngeal artery.
Loss of bony segments from vascular ischemia and subsequent aseptic necrosis is still a potential complication of complete maxillary osteotomy. Great care must be taken to avoid violating basic biologic principles and proper surgical technique. Poor incision design or extensive detachment of palatal mucosa are two actions that may deny proper perfusion to the mobilized segment. Excessive pressure by palatal splints, excessive and/or continued manipulation of the surgical site, and traumatic manipulation of the maxilla during downfracture are all potential causes of postoperative complications. Particular care must be taken in treatment planning for patients with compromised vascular supply from previous surgery and scarring (e.g., patients with cleft palate) to avoid aseptic necrosis.
Histology
Histologic examination of the surgical sites demonstrates transient ischemia followed by formation of a fibrinous clot between and around the osseous segments. Groups of red blood cells, with occasional white blood cells, are entrapped in this fibrin network. Approximately 1 week postoperatively an increased periosteal and endosteal vascular supply is seen, limiting osseous and pulpal ischemia. The space between the bony segments is filled with granulation tissue composed of fibroblasts and capillaries ( Figure 2-5 ).
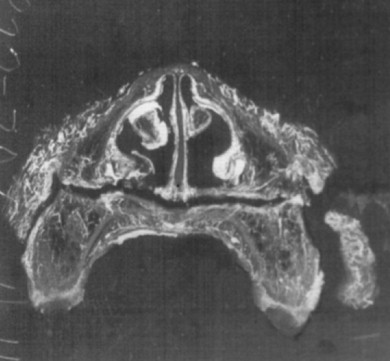
The tissue matures at 2 weeks and is composed of numerous blood vessels, with early signs of osteophytic bone arising from the periosteum and bony edges. Some evidence shows blood vessels connecting the bony segments. These blood vessels arise by endosteal proliferation. Some evidence of reattachment of soft tissue to underlying bone and the anastomoses of endosteal and periosteal vessels also has been found. Finally, a well-vascularized and viable dentition is seen.
The proliferation of endosteal vessels restores significant circulation between the bony segments at approximately 4 weeks. The segmental gap is filled with a bony callus composed of fibrous tissue and immature bone that demonstrates active osteoblastic activity ( Figure 2-6 ). The osteoid continues to mature until bony bridges connect the osseous segments at approximately 6 weeks postoperatively. The maturation of soft and bony tissue continues at the surgical site and appears similar to that of nonsurgical sites at 12 weeks.
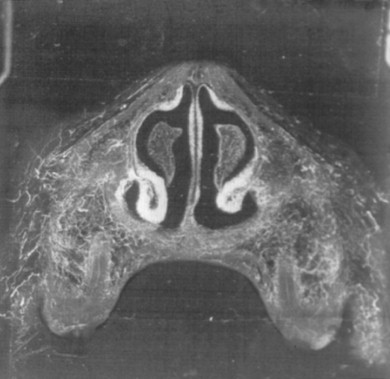
Gingival Tissue
Studies by Bell et al in the early 1970s used histologic and angiographic techniques to evaluate preoperative and postoperative vascular supply to the maxillary gingiva and dentition. Their results demonstrated transient ischemia with almost no clinical significance if the maxilla remained pedicled to the palatal and buccal gingiva. More recent investigations have used Doppler flowmetry for evaluation during and after maxillary osteotomies. Gingival blood flow is significantly decreased during surgery; however, minimal flow is maintained and is not significantly affected by ligation of the descending palatine arteries. Another study monitored the effect of local anesthesia with vasoconstrictor on intraoperative gingival blood flow. Dodson et al did find a significant reduction in gingival blood flow during the early phases of surgery, which could potentially add to the risk of detrimental ischemia. Other factors yet to be extensively studied include prolonged hypotensive anesthesia, age, gender, osseous segmentation, and direction and magnitude of segmental movement.
Dental Pulpal Blood Flow and Sensitivity
On the basis of their findings with microangiography to demonstrate revascularization of all tooth pulp chambers except those transected during osteotomy, Bell et al postulated that an osteotomy distance of 5 to 6 mm from the apices of the teeth is necessary to ensure tooth vitality. Further studies have used Doppler flowmetry to evaluate pulpal blood flow (PBF) before and after surgery as well as thermal and electric stimulation to evaluate the dental pulp sensitivity after surgery. Pepersack confirmed that if bony cuts are placed 5 to 6 mm away from the apex of the root, tooth loss is minimal. Sugg et al supported these findings of minimal loss of tooth vitality.
Variability exists in the reports regarding PBF in the postoperative period. Emshoff et al and Sato et al both found significantly decreased PBF postoperatively with a slow trend increasing back to near presurgery flow at 6 months to 1 year. Whereas Ramsay et al, Buckley et al, and Justus et al reported a hypervascular period 1-3 weeks post op with increased PBF relative to baseline. The clinical significance of peri-operative PBF is unclear. Regardless of the PBF in the short term after surgery, the long-term (>12 months) recovery of pulpal sensitivity approaches 100% as long as osteotomies are placed 5 to 6 mm from the apices of the teeth.
Maxillary Sinuses
Unfortunately few studies have investigated the effect of maxillary osteotomy on sinus disease. The incidence of postoperative sinonasal disease appears to be relatively low and, in fact, many patients with preoperative sinus disease may improve. Kahnberg and Engström 53
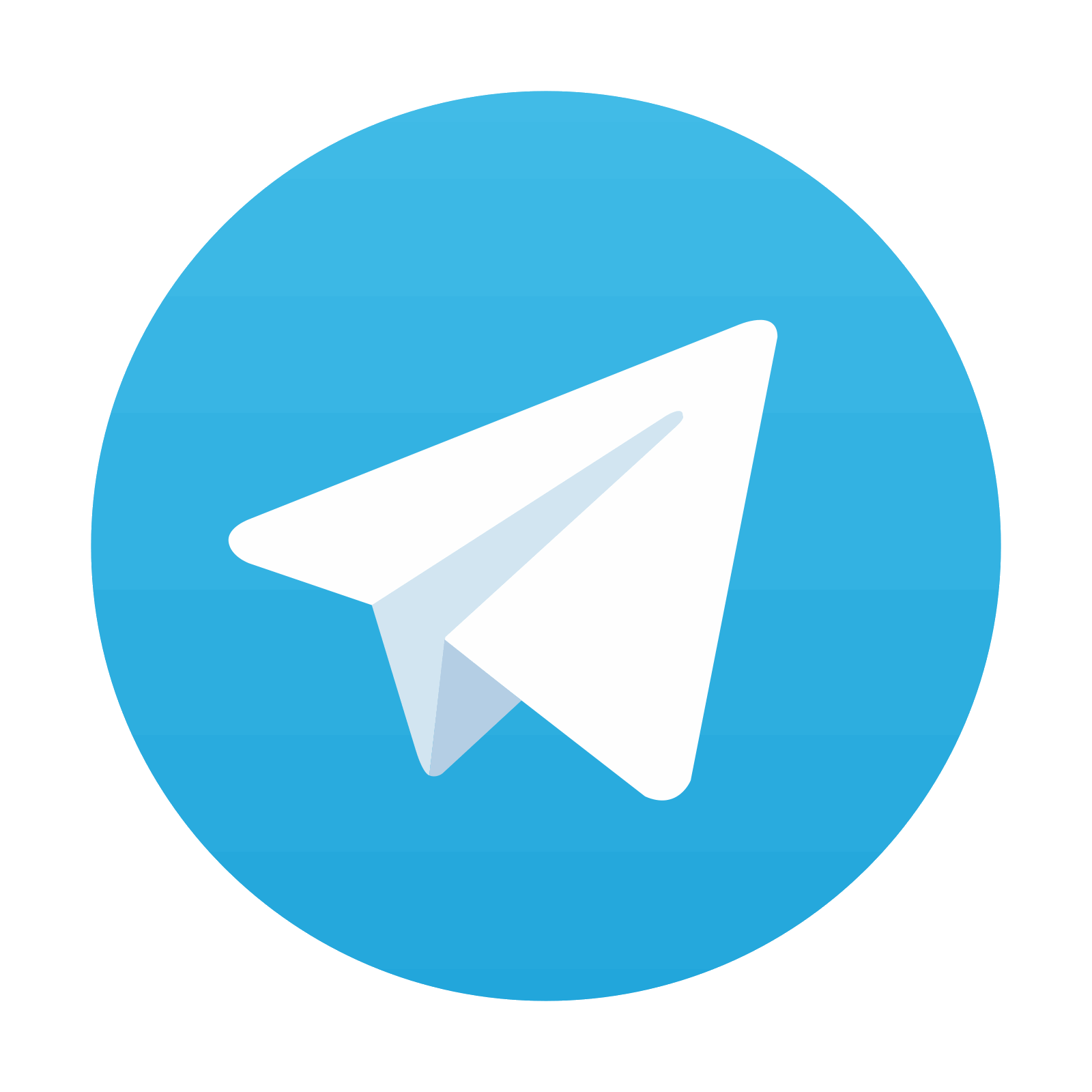
Stay updated, free dental videos. Join our Telegram channel

VIDEdental - Online dental courses
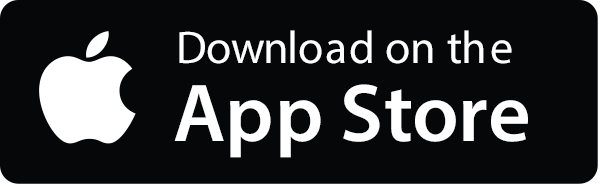
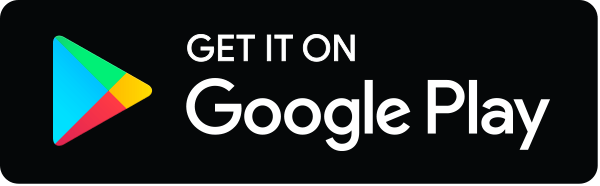