Fig. 7.1
Scanning electron micrograph of anodized TiUnite implant surface. The typical porous surfaces (octopus-trap-like feature) indicate that the sparking has occurred to form oxide layer
Figures 7.2 and 7.3 show the results of the chemical analyses of the anodized oxide layer of the TiUnite implant by means of cross-sectional transmission electron microscopy and energy-dispersed X-ray (EDX) spectroscopy. The surface has large undulations, and the thickness of the oxide layer is estimated to be approximately 3–10 μm. A large crack and a pore can be observed between the titanium base and the implant surface in Fig. 7.2. A protrusion and a depression are evident on the surface. Note that the depression located at the left top corner of implant surface (squared box). The dark-field transmission electron micrograph of the depression on the surface indicated that the left half of the micrograph shows an amorphous contrast, while the right half has crystalline structure. The diffraction patterns and EDX spectra taken from points b and c in Fig. 7.3a are shown in Fig. 7.3b, c. The diffraction pattern from the amorphous phase (point b) has halos. In contrast, the diffraction pattern taken from point c shows that the small crystals represent the TiO2 phase of the anatase-type structure. The size of the anatase crystal is estimated to be around 10 nm. In the EDX spectra measured from the amorphous phase (Fig. 7.3b), a strong phosphorus peak was detected, which is considered to be derived from a phosphate anion (P) in a phosphoric solution during anodization process. The phosphate anion from the electrolyte is incorporated and concentrated into the amorphous phase of the oxide layer. Previous reports using XPS and AES [22, 32, 33] revealed similar results that P was incorporated into anodic oxide surfaces, whereas XRD study indicated that the titanium phosphates were identified in anodic oxide anodized in H3PO4 electrolyte (1 mol/l) at electrical potentials between 100 and 250 V [34]. Previous in vitro studies suggested that the phosphate incorporation into anodic surface oxides on titanium and its alloys has beneficial effect that is capable of precipitation of bioactive Ca–P compounds during immersion in simulated body fluids (SBF) [35–38]. Sul et al. [39] demonstrated that the implant anodized in phosphoric acid showed strong bone reaction and provided direct chemical bonding sites for calcium ions and hydroxyapatite of the bone matrix during biologic mineralization.
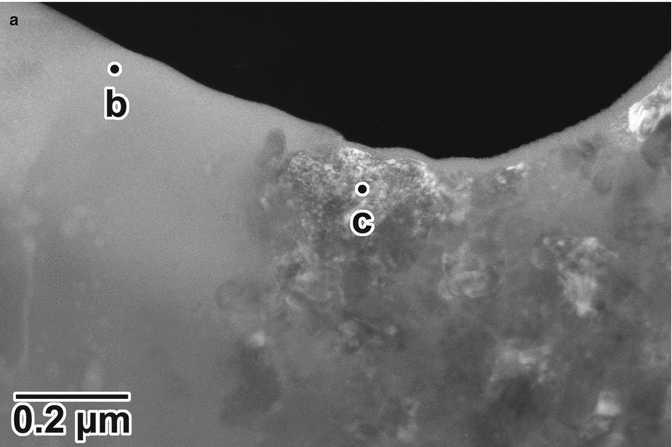
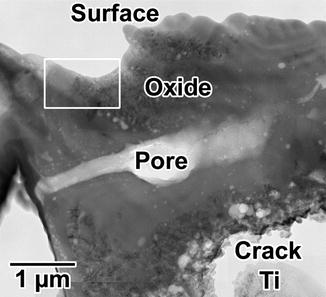
Fig. 7.2
Low-magnification transmission electron micrograph of the TiUnite surface. The upper portion of the photo shows the implant surface
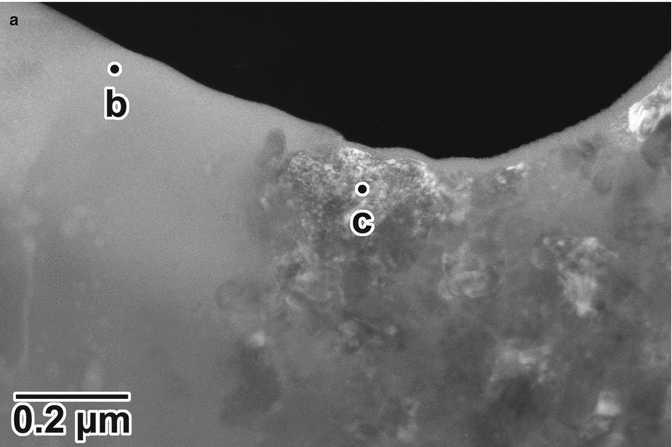
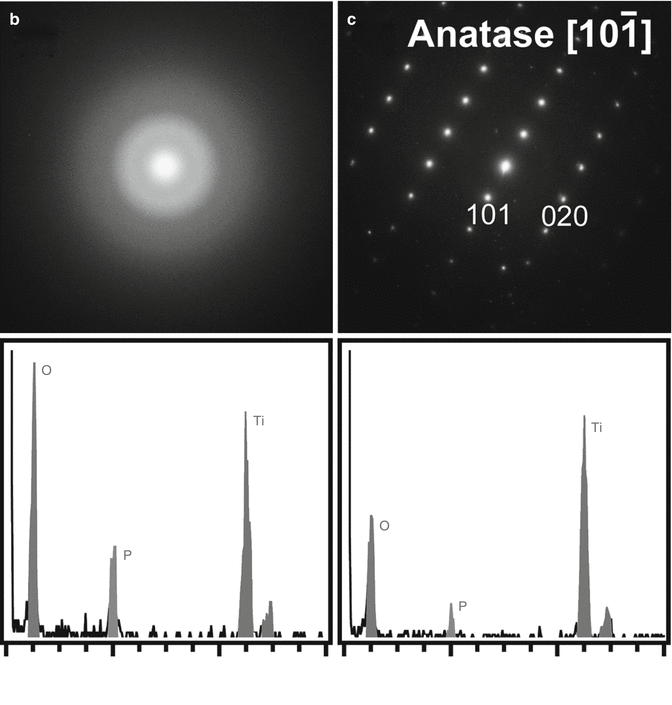
Fig. 7.3
(a) Dark-field transmission electron micrograph of the depression (squared box) on the surface shown in Fig. 7.2. Diffraction patterns and energy-dispersed X-ray spectra measured from points (b, c) in (a)
The elemental ions contained in the electrolyte are usually present in the thick, porous ASD film oxide, and the ion concentration decreases from the outer layer inward the bulk substrate [40]. For example, phosphorous ion was found to be embedded in titanium oxide layer after anodization with a H3PO4 electrolyte [41], and its concentration decreased from outer surface inward the bulk substrate. Another approach to make the anodized titanium bioactive was reported to introduce apatite layers onto the surface. In SBF, the spark-discharged anodized titanium oxide could induce apatite formation on its surface [25]. One advantage of this method is that the composition and surface morphology of the resulting apatite layer is very similar to those of the apatite in natural bone. In the studies conducted by Ishizawa et al. [42, 43], c.p. titanium anodized in an electrolytic solution containing sodium beta-glycerophosphate (beta-GP) and calcium acetate (CA) formed a thin calcium phosphate layer having a Ca/P ratio equivalent to hydroxyapatite (HA) in natural bone. In these studies, the thin Ca–P layer formed on the anodized substrates subjected to the hydrothermal treatment at 300 °C to form HA crystals with fully coverage the surface.
Biological Properties of Anodized Titanium
In Vitro Studies
Numerous studies were carried out regarding the cell compatibilities on anodized titanium and its alloys surface. Especially, since these materials are supposed to be used for the bone-anchored apparatuses in the field of orthopedic and dental implant, bone cells such as osteoblast or bone marrow stromal cells were investigated for cell attachment, proliferation, and differentiation. In essence, there are two factors to influence the cell compatibilities for anodized titanium oxide. One is the special morphology with the micro- and nanopores, and another is the effect of surface chemistry of the anodic oxidized layer.
Rodriguez et al. [44] investigated in vitro osteoblast response to anodized titanium (A) and anodized titanium followed by hydrothermal treatment (AH). Due to the deposition of calcium and phosphorus ions on titanium oxide during anodization, the grown apatite-like crystals were observed after AH. Enhanced cellular function and mineralization, as indicated by total protein synthesis and osteocalcin production, respectively, were also observed after AH. Consequently, the phenotypic expression of osteoblast was enhanced by the presence of calcium phosphate or apatite-like crystals on anodized or hydrothermally treated Ti surfaces [45]. Zhu et al. [40, 46] investigated the effects of topography and composition of anodized titanium surfaces on cell behavior of osteoblast [40]. When the anodic oxidation was carried out in two kinds of electrolytes, H3PO4 or Ca–GP and CA, the P or Ca and P were incorporated into the anodized surfaces, respectively. Cell culture experiments demonstrated non-cytotoxicity and an increase of osteoblast adhesion and proliferation by the anodic oxides. Osteoblast cells on the surfaces with micropores showed an irregular and polygonal growth and had many lamellipodia, while osteoblasts on the smooth titanium surface or on anodic oxides formed at low voltages showed many thick stress fibers and intensive focal contacts. Therefore, porous structures with micrometer order supply positive guidance for anchorage-dependent cells to attach, leading to enhanced cell attachment. In contrast, the cells are attached to a smooth titanium surface by focal contacts as predominant adhesion structures.
The submicron-ordered structures are also important variables in determining osteoblast response to substrate topography [47]. Growth behavior of human osteoblast cell on control smooth and anodized titanium surfaces with a nonporous structure was studied using an osteoprecursor cell line [48]. Cell adhesions and differentiation on anodized surfaces were enhanced with vinculin protein and alkaline phosphatase production. The 3-(4,5-dimethylthiazol-2-yl)-2,5-diphenyl tetrazolium assays also showed an increase in living cell density and proliferation with anodized surfaces. It was obvious that nanosized rough surface morphology was one of the important factors to achieve good cell/materials interaction.
In addition to bone cell behaviors, the collagen fiber which is the major bone matrix was tightly immobilized and partially incorporated into the anodic oxide layer in SBF [30]. The geometry of type I collagen is a triple helix with 300 nm in length and 0.5 nm in width and has a periodicity of 67 nm. On the other hand, HA crystal is approximately 20–40 nm in length and deposits at the periodic gap of the collagen fiber [49]. This indicates the theoretical importance of nanosized topography of biomaterial surface.
Li et al. demonstrated the bioactivity and the biocompatibility of anodized nano-structure of the Ti by SBF soaking test and in vitro cell culture test, respectively [50]. The formation of nanostructures induced hydrophilicity, and bone-like apatite formation resulted in enhancement of cell adhesion and proliferation on the anodic oxidation. Surface energy and hydrophilicity were known to play an important role in subsequent cellular responses on biomaterials. Kim et al. [51] reported a test for Ti discs with two different surface topographies (machined and anodized), the surface energy, surface wettability, and osteoblast responses, including cell attachment capacity, cell proliferation rate, and cell differentiation level, significantly increased on anodized Ti surfaces immersed in modified SBF. The effects of biomimetic deposition with modified SBF on physiochemical surface characteristics and cell biological responses were greater on anodized surfaces than on machined surfaces.
Interestingly, Giordano et al. [52] demonstrated that the anodization treatment of pure titanium (applied voltage, 130 V) and Ti6Al4V alloy (applied voltage, 120 V) with higher voltage, compared to the untreated c.p. titanium and Ti6Al4V and those anodized with low voltages, resulted in a greater decrease in bacterial attachment and biofilm formation in both in vitro and in vivo experiments. In contrast, the anodization with high voltages was found to promote osteoblast and fibroblast proliferation. These observations indicated that the anodization treatments with high voltages may contribute to preserve the tissue integration and to reduce bacteria colonization on titanium and titanium alloy for implant applications. Kang et al. [53] also indicated the antibacterial effect and cytocompatibility of anodized TiO2 film that had nanostructures and contained Cl. They carried out two-step anodization, where a nanostructured titanium oxide film was formed by anodization in hydrofluoric acid and followed by NaCl solution. The Cl atoms were confirmed to incorporate into the coatings. The cell wall of the bacteria might be destroyed due to the antibacterial effect of Cl.
In Vivo Studies
The promising results from in vitro studies require conformations by in vivo studies to fully understand the relevance of anodization. From the survey of in vivo investigations, the clinical deployment was intended. Therefore, the screw-shaped implants with the size of clinical use were fabricated, and the efficacy of the surface modification was investigated by either histomorphometric analyses such as bone-to-implant contact and bone area around the vicinity of the implant or biomechanical tests such as pushout test and removal torque measurement. The surface modification of the titanium implant for in vivo studies was either spark anodization or spark anodization with hydrothermal treatment; hence, most studies provided similar porous surface morphology and their average roughness was 0.82–1.97 μm. These numbers are categorized as moderately roughened surface due to the high voltage of anodization. However, the surface chemical composition (mainly titanium oxide containing HA, P, S, and Ca) varied widely depending on the electrolyte used. Ishizawa and coworkers [42, 43] focused on anodized titanium enriched with Ca–P, and thin HA film (1–2 μm) was produced as described in in vitro study. The strong bone bonding was confirmed by pushout tests after 8 weeks of implantation into rabbits. Additionally, the histomorphometric analyses indicated much bone formation with anodized and hydrothermal-treated implant. Son et al. reported no significant difference in the percent bone contact for all samples but did find significantly increased removal torque strength for anodized implants after 6 weeks of implantation into a rabbit [54]. The chemical bonding between bone and deposited HA would be suggested. The electrolyte mixed with H3PO4/H2SO4 was introduced by several researchers [55–57]. Either special feature of tubular structure with interchanneled pores or incorporated P and S chemistry would provide significantly high pushout strength in the rabbit model.
A series of studies regarding anodized implant have been published by Sul and coworkers [56–59]. They systematically tried to prepare S-, P-, and Ca-incorporated implants by anodization with similar surface morphology and roughness. Prepared implants were inserted in the femora and tibiae of mature New Zealand white rabbits for 6 weeks [35]. Significantly higher removal torque value was shown in Ca-containing and S-containing anodized titanium implants compared to non-anodized titanium implants. The bone-to-implant contact was 186, 232, and 272 % higher in S, P, and Ca implants, respectively, when compared to the control groups. These results indicated that the ions incorporated into the titanium oxide layer during anodization had important roles in enhancing bone formation. Subsequently, magnesium (Mg)-incorporated anodic oxidized titanium implants were investigated in the same model. The result showed that the Mg-incorporated titanium implants significantly improved bone responses as compared with machine-turned control implants. When the differences and similarities of the surface oxide properties of controls and experimental implants were considered, the enhanced bone responses of Mg-incorporated implants could be explained by the Mg surface chemistry of the experimental implants [58].
Intriguingly, we found that anodized porous TiO2 implants acquire photoinduced hydrophilicity when irradiated with ultraviolet (UV) light [31]. The water contact angle for the ordinary anodized porous TiO2 implants (TiUnite) was 44°, whereas it dramatically decreased to 11° after 24 h of UV irradiation, which indicates that the anodized porous TiO2 implants have inherent photoinduced hydrophilicity. However, no significant enhancement of bone regeneration around the anodized porous TiO2 implants irradiated with UV could be seen after 4 weeks of healing in the rabbit tibiae. Subsequently, further improvement of the photoinduced hydrophilicity of the anodized porous TiO2 implant was attempted by fluoride modification [59]. The result showed that the anodized porous TiO2 implants modified with fluoride demonstrated significantly greater degrees of bone-to-metal contact than control implants after 2 and 6 weeks of healing. These results proved that the enhanced photoinduced hydrophilicity of the anodized implants modified with NH4HF2 promoted bone apposition during early stages of osseointegration.
It is noteworthy that plaque accumulation and subsequent periimplantitis are suspected to be due to the rough surfaces with pore structure of anodized surface. Albouy [60] alerted to take precautions against more plaque accumulation and periimplantitis progress of anodized TiUnite implant than machine-turned implant in the ligature-induced periimplantitis dog model. Clinical verification would be necessary to clarify the risk of acquiring periimplantitis on anodized implant.
Anodized Implant: Clinical Trials
The most well-known anodized commercial dental implant is TiUnite which is spark anodized in H3PO4-containing electrolyte. The surface properties of the oxide layer were characterized as several micrometer thickness and porous topography with 4–5 μm-sized pores; chemical elements containing Ti (15 %), O (55 %), C (20 %), P (5 %), S (1 %), and Si (1 %); the presence of anatase and rutile crystal of TiO2, and an average roughness (Ra) of approximately1.2 μm [61
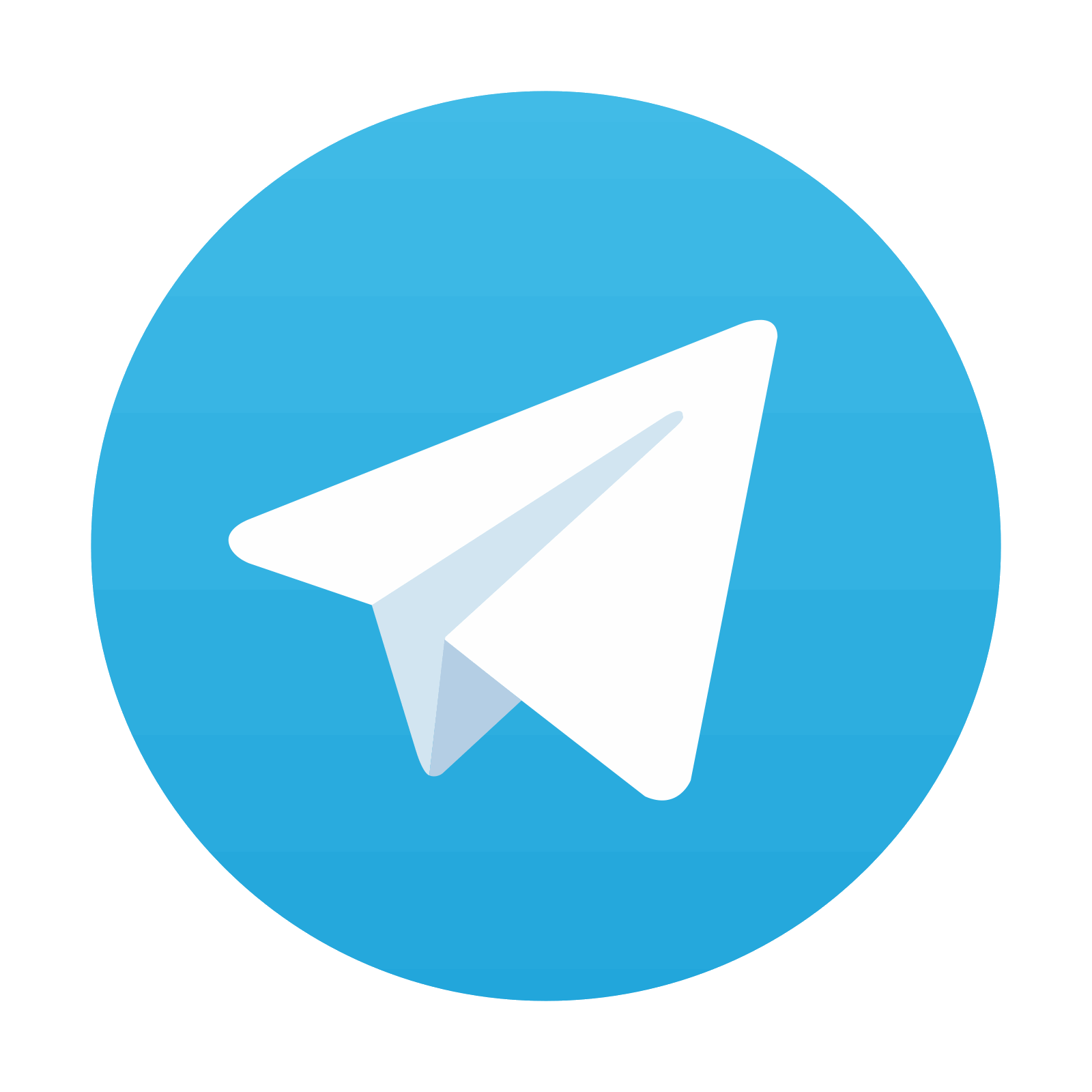
Stay updated, free dental videos. Join our Telegram channel

VIDEdental - Online dental courses
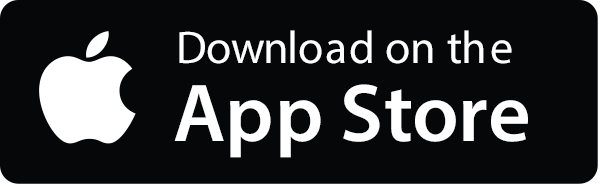
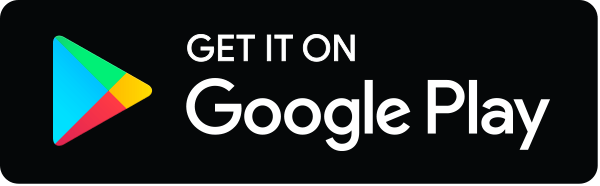