Abstract
Periodontitis is a major chronic inflammatory disorder that can lead to the destruction of the periodontal tissues and, ultimately, tooth loss. To date, flap debridement and/or flap curettage and periodontal regenerative therapy with membranes and bone grafting materials have been employed with distinct levels of clinical success. Current resorbable and non-resorbable membranes act as a physical barrier to avoid connective and epithelial tissue down-growth into the defect, favoring the regeneration of periodontal tissues. These conventional membranes possess many structural, mechanical, and bio-functional limitations and the “ideal” membrane for use in periodontal regenerative therapy has yet to be developed. Based on a graded-biomaterials approach, we have hypothesized that the next-generation of guided tissue and guided bone regeneration (GTR/GBR) membranes for periodontal tissue engineering will be a biologically active, spatially designed and functionally graded nanofibrous biomaterial that closely mimics the native extra-cellular matrix (ECM).
Objective
This review is presented in three major parts, including (1) a brief overview of the periodontium and its pathological conditions, (2) currently employed therapeutics used to regenerate the distinct periodontal tissues, and (3) a review of commercially available GTR/GBR membranes as well as the recent advances on the processing and characterization of GTR/GBR membranes from a materials perspective.
Significance
Studies of spatially designed and functionally graded membranes (FGM) and in vitro antibacterial/cell-related research are addressed. Finally, as a future outlook, the use of hydrogels in combination with scaffold materials is highlighted as a promising approach for periodontal tissue engineering.
1
Introduction
The aging population is one of the main reasons we need to advance the understanding of dental biomaterials. There will be a considerable increase in the number of surgical and restorative procedures related to either oral rehabilitation with the placement of dental implants and ceramic crowns or to periodontal regeneration, which often requires the use of membranes for guided tissue/bone regeneration (GTR/GBR) and grafting materials.
Periodontitis is one of the most destructive pathologies that affects the integrity of the periodontal system and lead to damage of the periodontal tissues and, ultimately, tooth loss . Data from the National Institute of Dental and Craniofacial Research (NIDCR, National Institutes of Health, United States) has revealed that nearly 90% of adult populations more than 70 years old present at the least a moderate level of periodontal-related diseases . Indeed, several research findings have suggested an association of periodontitis with systemic disorders, such as diabetes, cardiovascular and respiratory diseases .
It is well known that clinical and/or surgical interventions are a mandatory step in the reestablishment of the health of the periodontal tissues. During the last decade, various regenerative surgical modalities have been suggested and examined for the regeneration of periodontal specific tissues; i.e., alveolar bone, cementum, periodontal ligament and gingiva . These treatments have encompassed the utilization of a wide variety of surgical approaches, barrier membranes, a series of bone grafts and other osteoconductive/inductive materials or protein mixtures, exogenous growth factors, cell-based technology and genes from recombinant technology. Among these approaches, periodontal tissue regeneration has met with considerable success following the use of GTR/GBR strategies in certain, well-selected clinical cases. Nonetheless, results are variable and reliant on patient age, defect size, generics, and other demographic and lifestyle effects .
In the early 1990s, Langer and Vacanti proposed a tissue engineering route to regenerate lost tissue and restore the various functions of damaged human tissues and organs . The principles of tissue engineering for regeneration involve the combination and interplay of three major elements such as scaffolds or membranes, regenerative cells or stem cells, and cell signaling molecules or growth factors ( Fig. 1 ).

Advances in the science and technology of nanomaterials have led to increased enthusiasm for approaches such as electrospinning (e-spinning) of biomimetic multifunctional growth-enhancing regenerative membranes for periodontal tissue engineering. Electrospun nanofibrous scaffolds/membranes mimic more closely the scales and morphologies of the extracellular matrix (ECM) proteins (fibers with diameters ranging from 50 to 500 nm).
To provide a comprehensive update on progress in the field, this review is arranged into three major parts, including (1) a brief overview of the periodontium and its pathological conditions, (2) currently employed therapeutics for use in the regeneration of the distinct periodontal tissues, (3) a critical review of commercially available GTR/GBR membranes and the most recent advances in the processing and characterization of GTR/GBR membranes from a materials perspective. A special emphasis on spatially designed and functionally graded membrane (FGM) and in vitro antibacterial/cell-related studies is provided. Finally, as a future outlook, the science of hydrogels as it applies to periodontal bone tissue regeneration is also included.
2
Periodontal diseases and treatment modalities
Periodontitis affects the integrity of the periodontal tissues. The periodontium is an intricate system constituted of root cementum, periodontal ligament (PDL), alveolar bone, and the dentogingival junction ( Fig. 2 ). The periodontium anchors teeth to both the mandible and maxilla, preserves their position, and provides nourishment to the teeth. Additionally, it assures proper function and dissipation of forces, preventing injury to teeth, mandible, and maxilla. The cementum is an avascular as well as non-innervated calcified connective tissue that covers the root dentin and anchors periodontal ligament (PDL) fibers. The PDL is composed of collagen fibers and is responsible for absorbing/distributing functional forces to the alveolar bone. On one side, the PDL interfaces with root cementum and on the other with alveolar bone . The alveolar bone supports the teeth and the gingival tissues – it distributes and absorbs mastication forces through the PDL. The performance of the periodontium depends upon its structural integrity and interactions among its components . However, periodontal disease can progressively destroy the periodontium, ultimately resulting in tooth loss. Initiated and maintained by bacterial stimulation of a chronic inflammatory and immune response, tissue integrity is compromised with the loss of soft tissue attachment to the root surface. Loss of alveolar bone results in the formation of a periodontal pocket around the tooth that acts as a reservoir supporting the growth of anaerobic bacteria (e.g., Porphyromonas gingivalis and Prevotella intermedia ), which ultimately may lead to tooth loss .

Gingival recession is another periodontal problem that affects millions of people worldwide . It is a multifactorial condition (i.e., periodontal disease, trauma, gender, and tobacco consumption) found primarily in adult patients regardless of oral hygiene . It is a result of apical migration of the gingival margin that leads to root surface exposure .
Different treatment modalities have been suggested in order to regenerate the periodontal tissues damaged in cases of both gingival recession and periodontitis. To treat cases of gingival recession, traditional surgical procedures use transplanted autogenous tissue (i.e., from the palatal area). Tissue-derived collagen-based membranes have started to replace this procedure, which avoids multiple surgical sites operation, so diminishing considerably the associated pain and morbidity . As mentioned earlier, the strategy of using either synthetic or tissue-derived membranes as barriers with or without calcium phosphate-based bone graft materials has received great attention for restoring the function of a damaged or pathologically affected periodontium .
2
Periodontal diseases and treatment modalities
Periodontitis affects the integrity of the periodontal tissues. The periodontium is an intricate system constituted of root cementum, periodontal ligament (PDL), alveolar bone, and the dentogingival junction ( Fig. 2 ). The periodontium anchors teeth to both the mandible and maxilla, preserves their position, and provides nourishment to the teeth. Additionally, it assures proper function and dissipation of forces, preventing injury to teeth, mandible, and maxilla. The cementum is an avascular as well as non-innervated calcified connective tissue that covers the root dentin and anchors periodontal ligament (PDL) fibers. The PDL is composed of collagen fibers and is responsible for absorbing/distributing functional forces to the alveolar bone. On one side, the PDL interfaces with root cementum and on the other with alveolar bone . The alveolar bone supports the teeth and the gingival tissues – it distributes and absorbs mastication forces through the PDL. The performance of the periodontium depends upon its structural integrity and interactions among its components . However, periodontal disease can progressively destroy the periodontium, ultimately resulting in tooth loss. Initiated and maintained by bacterial stimulation of a chronic inflammatory and immune response, tissue integrity is compromised with the loss of soft tissue attachment to the root surface. Loss of alveolar bone results in the formation of a periodontal pocket around the tooth that acts as a reservoir supporting the growth of anaerobic bacteria (e.g., Porphyromonas gingivalis and Prevotella intermedia ), which ultimately may lead to tooth loss .
Gingival recession is another periodontal problem that affects millions of people worldwide . It is a multifactorial condition (i.e., periodontal disease, trauma, gender, and tobacco consumption) found primarily in adult patients regardless of oral hygiene . It is a result of apical migration of the gingival margin that leads to root surface exposure .
Different treatment modalities have been suggested in order to regenerate the periodontal tissues damaged in cases of both gingival recession and periodontitis. To treat cases of gingival recession, traditional surgical procedures use transplanted autogenous tissue (i.e., from the palatal area). Tissue-derived collagen-based membranes have started to replace this procedure, which avoids multiple surgical sites operation, so diminishing considerably the associated pain and morbidity . As mentioned earlier, the strategy of using either synthetic or tissue-derived membranes as barriers with or without calcium phosphate-based bone graft materials has received great attention for restoring the function of a damaged or pathologically affected periodontium .
3
Periodontal specific tissue engineering
The different strategies of periodontal therapy aim to (1) reduce and/or eliminate inflamed tissues caused by bacterial plaque, (2) correct defects or anatomical problems due to the disease, and (3) regenerate new periodontal tissues . Development of new cementum with PDL fibers connected to alveolar bone is the main goal of periodontal regeneration . Two surgical techniques have been increasingly used to restore/regenerate the different periodontal tissues, namely (1) guided tissue regeneration (GTR) and (2) guided bone regeneration (GBR) .
The use of an occlusive membrane interfacing with gingival connective tissue/epithelium and a PDL/alveolar bone tissue to promote periodontal tissue regeneration is called GTR. An occlusive membrane acts as a barrier when placed into the surgical site, preventing connective and epithelial tissue migration into the defect. Progenitor cells located in the remaining periodontal ligament, adjacent alveolar bone, or blood are then able to recolonize the root area and differentiate into a new periodontal supporting apparatus with the formation of new bone, PDL, and cementum. Another important application of the concept of guided regeneration concerns the restoration of deficient alveolar sites (e.g., an extraction site and deficient alveolar ridge) for posterior implant placement. This process has been named guided bone regeneration (GBR). Effective results have been achieved when using membranes for the treatment of intrabony defects, furcations, as well as for the repair of marginal tissue recession defects . However, it seems so far that a consensus has not been reached in terms of the successful use of GTR membrane over connective tissue graft (CTG) in recession defects . Meanwhile, Wennstrom reported that GTR improved root coverage when compared to free gingival grant (FGG) and lateral sliding flap . A recent meta-analysis reported greater probing depth reduction and improved attachment level in guided tissue regeneration-treated sites compared with open-flap debridement . Indeed, a systematic review by Murphy and Gunsolley highlighted important clinical advantages promoted by guided regeneration procedures opposed to conventional open flap debridement for treating furcation and intrabony defects.
4
Barrier membranes for GTR and GBR applications
The strategy to isolate the periodontal defect with a mat-like material (resorbable or non-resorbable) that will function as a physical barrier to avoid gingival cell invasion led to the development of GTR/GBR membranes . These GTR/GBR membranes need to exhibit: (1) biocompatibility to allow integration with the host tissues without eliciting inflammatory responses, (2) proper degradation profile to match those of new tissue formation, (3) adequate mechanical and physical properties to allow its placement in vivo, and (4) sufficient sustained strength to avoid the membrane collapse and perform their barrier function . GTR/GBR membranes are divided into two groups, non-resorbable and resorbable, according to their degradation characteristics.
4.1
Stability/degradation characteristics of GTR/GBR membranes
The so-called “gold standard” non-resorbable ( Table 1 ) membranes for GTR/GBR procedures currently on the market are (1) high-density polytetrafluoroethylene, PTFE (e.g., Cytoplast ® TXT-200, Osteogenics Biomedical, Lubbock, TX, USA) ( Fig. 3 A ) and (2) titanium-reinforced high-density polytetrafluoroethylene (e.g., Cytoplast ® Ti-250, Osteogenics Biomedical, Lubbock, TX, USA) . PTFE membranes are inert and biocompatible, act as a cellular barrier, provide space for tissue regeneration, and allow tissue integration. It has been suggested that there is a favorable correlation between the level of bone regeneration and space protection . Studies have revealed that titanium reinforcement of high-density PTFE membranes ( Fig. 3 B) lead to superior regenerative capacity when compared to traditional expanded PTFE membranes mainly due to the additional mechanical support provided by the titanium frame against the compressive forces exerted by the overlying soft tissue . One of the disadvantages of non-resorbable membranes is the necessity of performing an additional surgery for their removal, which implicates not only additional pain and discomfort but also an economic burden.
Resorbability | Commercial name | Composition | Mechanical strength | Degradation rate | Biological properties |
---|---|---|---|---|---|
Non-resorbable | Cytoplast ® TXT-200 | High-density polytetrafluoroethylene (d-PTFE) | N/A | Non-degradable ® | Biocompatible |
Cytoplast ® Ti-250 | Titanium-reinforced high-density PTFE | N/A | Non-degradable ® | Biocompatible | |
Resorbable synthetic | Resolut LT ® | Poly- dl -lactic/co-glycolic acid | 11.7 MPa | 5–6 months | Biocompatible |
Vicryl ® | Polyglactin 910 | N/A | ∼9 months | Biocompatible | |
Polyglycolide/polylactide (9:1, w/w) | |||||
Atrisorb ® | Poly- dl -lactide and solvent (N-methyl-2-pyrrolidone) | N/A | 6–12 months | Biocompatible | |
Resorbable collagen-based | AlloDerm ® | Collagen Type-I derived from cadaveric human skin | 9.4–21.5 MPa | ∼16 weeks | Biocompatible |
Bio-Gide ® | Collagen derived from porcine skin (Types I and III) | 7.75 MPa | 24 weeks | Biocompatible | |
BioMend Extend ® | Collagen Type-I derived from bovine tendon | 3.5–22.5 MPa | 18 weeks | Biocompatible | |
Cytoplast ® RTM | Collagen Type-I derived from bovine tendon | N/A | 26–38 weeks ® | Biocompatible |
In order to eliminate the second surgical procedure, resorbable barrier membranes have been developed . Processing techniques based on either melting (i.e., polymer heated above the glass transition or melting temperature) or solvent casting have been used to fabricate polymer-based membranes for GTR/GBR applications. Solvent casting/particulate-leaching and phase inversion are common methods to fabricate porous, three-dimensional scaffolds and/or membranes for tissue engineering. In the solvent-casting/particulate-leaching method, a leachable porogen, usually an inorganic salt (e.g., sodium chloride or organic sugars) of the desired particle size is combined with a polymer solution in a mold. After solvent evaporation, the salt or sugar crystals are dissolved in water, which leaves a porous polymer structure . In this technique, the pore size and porosity level of the membrane/scaffold can be tailored by adjusting the particle size and the salt or sugar/polymer ratio, respectively. Unfortunately, organic solvents commonly used in this technique may negatively affect cell and tissue response upon implantation .
The majority of synthetic polymer resorbable membranes for periodontal regeneration on the market are either based on polyesters (e.g., poly(glycolic acid) (PGA), poly(lactic acid) (PLA), poly(ε-caprolactone) (PCL), and their copolymers) or tissue-derived collagens . The polyester-based membranes ( Table 1 ) are biocompatible, biodegradable, and easier to handle clinically when compared to PTFE membranes as well as allowing tissue integration. Their resorption rate is important since these membranes must function for at least 4–6 weeks to allow successful regeneration of the periodontal system . Generally, the biodegradation of these polyesters involves non-enzymatic cleavage of PGA and PLA into pyruvic and lactic acids, respectively, which are common end-products of carbohydrate digestion. Milella et al. evaluated both the morphological and mechanical characteristics of commercially available polyester-based membranes (i.e., Resolut ® LT and Biofix ® ) . Although the membranes demonstrated initially high strength (∼12–14 MPa), they completely lost their structural and mechanical properties within 4 weeks of incubation in culture medium. The maximum strength after 14 days of exposure decreased significantly (below 1 MPa) . In a recent study Li et al. processed membranes based on nano-hydroxyapatite/polyamide-66 with a porosity gradient and suggested that n-HAp addition guaranteed a membrane with good tensile strength (2–3 MPa). In addition, the reported poor cell response limits their use in GTR/GBR applications.
Collagen is a major constituent of natural extracellular matrix (ECM). Tissue-derived collagen-based membranes ( Table 1 ) from human skin (Alloderm ® , LifeCell, Branchburg, NJ, USA), bovine Achilles tendon (Cytoplast ® RTM Collagen, City, State, USA) or porcine skin (Bio-Gide ® , Osteohealth, Shirley, NY, USA) are important alternatives to synthetic polymers in GTR/GBR procedures due to their excellent cell affinity and biocompatibility . However, type-I collagen may have limitations in its use due to the high cost and poor definition of its commercial sources, which make it difficult to control degradation and mechanical properties. AlloDerm ® is an acellular freeze-dried dermal matrix graft ( Fig. 4 ) composed mainly of type-I collagen derived from human cadaveric skin . According to its manufacturer, this proprietary process does not cause damage to the critical biochemical structural cues needed to maintain the tissue’s natural regenerative properties and leaves behind an extracellular collagenous matrix that provides the basis for tissue structure and guides cellular functions .
Collagen-based membranes have shown very poor performance in vivo as the membrane starts to degrade . Additionally, the risks of disease transmission due to the use of human- or animal-derived collagen may pose regulatory or other limitations, such as religious beliefs, on its use. Biomechanical properties and collagen matrix stability can be enhanced by means of physical/chemical crosslinking, by ultraviolet (UV) radiation, genipin (Gp), glutaraldehyde, 1-ethyl-3-(3-dimethylaminopropyl) carbodiimide hydrochloride (EDC), among others. It has been reported that exogenous crosslinking agents such as Gp not only significantly increase the stability of collagen-based tissues but also reduce its antigenicity. The formation of supplementary inter- or intramolecular crosslinks within the collagen fibers enhances mechanical properties of biological tissues . Bottino et al. investigated how the addition of a natural crosslinking agent, genipin (Gp), into the AlloDerm ® rehydration protocol affects the mechanical properties and collagen matrix stability . A significant enhancement in tensile strength compared to control was observed when Gp exposure time was increased from 30 min to 6 h. Differential scanning calorimetry analyses revealed a considerable shift in the denaturation temperature for crosslinked samples, which coincides with the increase in the enthalpy of denaturation . This finding agrees with prior investigations on the use of a crosslinking agent to enhance the stabilization of collagenous matrices derived from different biological tissues .
The critical disadvantages of both PTFE-based non-resorbable (e.g., second surgery) and resorbable membranes, mainly those based on collagen (e.g., insufficient mechanical properties, unpredictable degradation profiles), have led to studies of alternate membrane materials. Several research groups have been investigating the possibility of using membranes with a functionally graded structure to maintain sufficient mechanical properties during service, predictable degradation rate, and bioactive properties . Bone formation would be stimulated by calcium–phosphate based nanoparticles or growth factors (e.g., BMP-2, TGF-β, among others) on the hard tissue/membrane interface , and bacterial colonization would be inhibited by antibacterial drugs delivered at the soft tissue/membrane interface .
4.2
Designed membranes for zone-dependent bioactivity
In recent years, many research groups have tried to design and develop GTR/GBR periodontal membranes with the requisite features and properties by combining natural and synthetic polymers. These studies prepared GTR/GBR membranes using film casting , dynamic filtration and e-spinning of synthetic (e.g., PCL) and/or natural (e.g., collagen, chitosan) polymers. The membranes have been prepared with or without therapeutic drugs , growth factors , and/or calcium phosphate particles . A material with the necessary mechanical, degradation and biological properties is still needed to guarantee GTR/GBR its best in vivo performance. The majority of these methods result in membranes with very low clinical potential due to high density (i.e., difficulty in handling) and heterogeneity (i.e., non-uniform degradation rate).
The e-spinning technique has demonstrated great potential for processing membranes for periodontal regeneration . E-spinning is a particularly promising technique for synthesizing biomimetic nanomatrices, including membranes for GTR/GBR applications.
4.2.1
Electrospinning (e-spinning) for membranes
Formhals first introduced electrospinning or e-spinning in 1938 . Recently, numerous research groups have explored its use to generate fibrous scaffolds for tissue regeneration . A typical electrospinning apparatus includes a polymer solution/melt in a syringe, charged through a high voltage supply, and a grounded plate positioned at a predetermined distance from the tip of the needle ( Fig. 5 ). The potential difference overcomes the surface tension of the fluid droplet at the tip of the metal needle, which in turns results in the formation of the so-called Taylor cone. The fluid jet experiences whipping instabilities and tends to dry and form fibers with an average diameter ranging from several microns to tens of nanometers . Processing parameters including voltage, distance from tip to collector, collector type (rotating or static), solution properties (e.g., concentration, viscosity and conductivity) and flow rate present a great influence on fiber formation and morphology . The solution must be sufficiently concentrated so that the polymer chains are continuous and entangled and of suitable viscosity so a droplet can be maintained and the solution can be pumped through the syringe . The resulting mat can be composed of biocompatible and degradable natural or synthetic polymers or blends and normally resembles the arrangement of the native extracellular matrix (ECM). The fibers can be collected at a random orientation when using a static collector or with high degree of alignment by using a rotating mandrel .
Maintenance of wound stability is a key factor in attaining a successful outcome in regenerative periodontal surgery . Essentially, the three-dimensional (3D) structure shown by these e-spun membranes, with a high surface area of improved hydrophilicity and wettability, endow the structure with mechanical support and regulate cell functions guiding new bone formation into the defect . Li et al. have cultured different cells such as fibroblasts, cartilage cells, and mesenchymal stem cells on PLGA and PCL nanofibrous e-spun scaffolds and demonstrated the ability of the nanofiber structure to support cell attachment and proliferation . Because of the inherent high surface area, surface functional groups, interconnected pores and nano-scaled size, nanofiber-based scaffolds are more favorable than micro-fibers or any other morphological forms. In fact, nanofibrous scaffolds stimulate positive cell–ECM interactions, increase the proliferation rate, maintain cell phenotype, support differentiation of stem cells, support in vivo-like three-dimensional matrix adhesion and activate cell-signaling pathways by providing physical and chemical stimuli to cells . Systematic reviews on the e-spinning process and applications of these nanofibers in tissue engineering can be found elsewhere .
Sequential spinning or multi-layering is usually employed to fabricate scaffolds or membranes with different layer-structures. Sequential e-spinning of different polymers, both synthetic polymers alone and in combination with natural proteins enhances the mechanical-integrity and dimensional-stability of e-spun meshes . Synthetic/protein layer-by-layer membranes can also be fabricated by e-spinning in combination with freeze-drying or phase separation processes .
4.3
Functionally graded and multilayered membranes
GTR/GBR membranes for periodontal regeneration can be considered an interface-implant, which interfaces with gingival connective tissue/epithelium and a PDL/alveolar bone tissue. An interface-implant needs to utilize a graded-structure with compositional and structural gradients that meet the local functional requirements to engineer new periodontium by enhancing bone growth while preventing the gingival tissue down-growth. With this in mind, fabrication of a functionally graded three-layered membrane from PLGA, collagen and nano-hydroxyapatite by a layer-by-layer casting method was reported earlier . The membrane was designed with one side constituted by 8% nano-carbonated hydroxyapatite/collagen/poly(lactic-co-glycolic) acid porous membrane allowing cell adhesion, and the opposite face with a smooth PLGA nonporous film.
E-spun functionally graded nanofibrous tubular scaffolds with distinct chemical compositions as well as tailored degradation and mechanical properties have been reported recently . The rationale of having a periodontal membrane with a graded-structure again relies on the principle that one can tailor the properties of the different layers to design a membrane that will retain its structural, dimensional and mechanical integrity long enough to enhance periodontal regeneration. Accordingly, a novel functionally graded membrane (FGM) was designed and fabricated via multilayering e-spinning ( Fig. 6 ). The FGM consists of a core-layer (CL) and two functional surface-layers (SL) interfacing bone (nano-hydroxyapatite, n-HAp) and epithelial (metronidazole, MET) tissues. The CL comprises a neat poly( d , l -lactide-co-caprolactone) (PLCL) layer surrounded by two composite layers composed of a gelatin/polymer ternary blend (PLCL:PLA:GEL). SEM images showing the morphological characterization of the FGM layers are seen in Fig. 7 . While most biodegradable polymers are rigid and brittle, PLCL possesses exceptional elastic properties, which are critical for periodontal membranes during in vivo placement. The mechanical properties of the CL are a major contributor to and, therefore, predictor of the in vivo mechanical performance of the present functionally graded membrane (FGM) for the required duration. Under hydrated conditions, CL presented high tensile strength (8.7 MPa) and tensile modulus (156 MPa) with strain at failure of 375%. No delamination was observed in the CL membrane, indicating that the compositionally-graded-layers remained intact under physiological conditions . Cross-section SEM images are given in Fig. 8 . The addition of two surface-layers rich in protein decreased the mechanical tensile properties in both dry and hydrated conditions. The FGM exhibited a tensile strength of 3.5 MPa and a tensile modulus of 80 MPa with a strain at break equal to 297% .
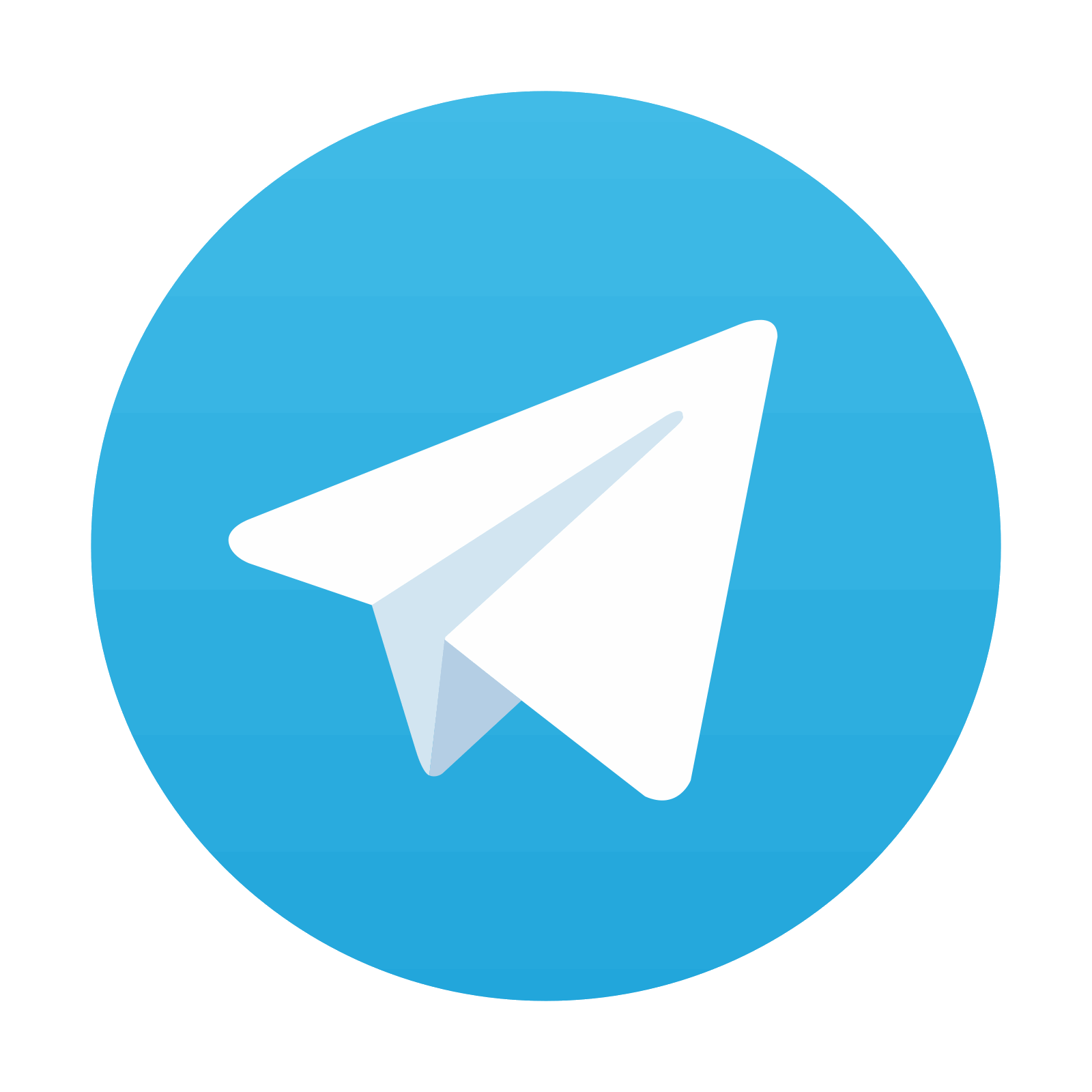
Stay updated, free dental videos. Join our Telegram channel

VIDEdental - Online dental courses
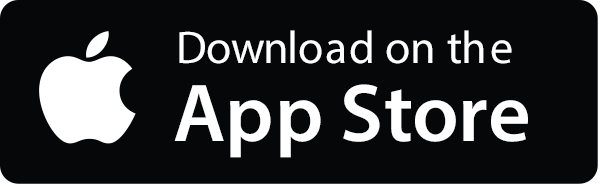
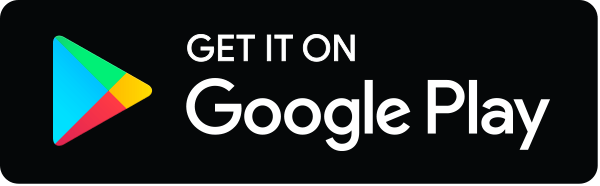
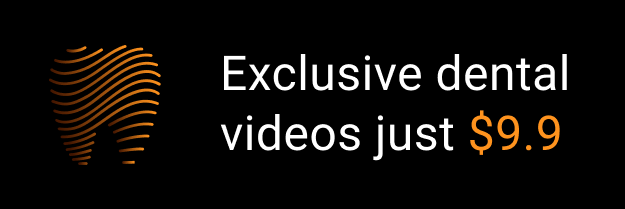