Fig. 12.1
Traditional classification of orthodontic implants (Courtesy T. Lietz)
Nomenclature
There is no general agreement on the nomenclature for this type of anchorage device. The names are based either on size (mini and micro), design (screw and plate), site of location (palatal and retromolar), or combinations thereof. Screw-shaped implants especially are named in more as 30 variations including mini-implant, mini-screw, micro-implant, and micro-screw [20]. The most used term is mini-implant. The term “micro” is not appropriate because the size of the screws is in the range of millimeters. These devices could be referred to as “mini” particularly compared to prosthodontic restorative implants as the diameter usually does not exceed 2 mm. The term “implant” is discussed in the light of different regulatory guidelines regarding classification of medical devices. These differences are important to consider when launching a product. Because the orthodontic implants are removed after use, they are not considered as “implants” according to the general ISO implant body definition (2.150, ISO 1942:2010 Dentistry – vocabulary): “[an implant] . . . …primary single component or portion of a dental implant which is intended to remain within the tissues.” However, the term orthodontic implant is included in the standard. Moreover, according to the Food and Drug Administration (FDA) in the USA and the European medical device regulatory within EU, an implant is “a device that is placed into a surgically or naturally formed cavity of the human body and is intended to remain there for a period of more than 30 days”. Because orthodontic implants always are used more than 30 days, they are according to this definition considered as “implants.”
Another frequently and popular term used for the various kinds of orthodontic implants are “temporary anchorage device.” This term, however, could be misleading because it is not accurate enough. Traditional anchorage appliances, including EOT and lingual arches, could also be referred to as temporary anchorage devices (TADs). Furthermore, and as discussed earlier temporary means less than 30 days, so the designation as such is not appropriate. Moreover, for nonspecialists, “palatal implants” could be mixed up with mini-implants placed in the palate medially or para-medially. These concerns and the fact that companies and scientists want to launch unique products with unique brand names will most probably make it difficult to agree on nomenclature. However, the suggested correct term for all these devices are orthodontic implants; subsequently, they can be classified according to design (plates, screws, or discs) and position. The nomenclature, however, needs to be further discussed. In this chapter, the most common terms for the orthodontic implants will be used (Fig. 12.1).
-
Palatal implant – placed mainly on the anterior palate
-
Onplant – placed only on the anterior palate
-
Mini-implant – placed inter-radicular in the mandible and maxilla and also in the palate
-
Mini-plate – placed on the body of the mandible or maxilla
Applications
The popularity of orthodontic implants, especially the mini-implants, stems from their ease of use with minimally invasive surgery as well as a high level of patient comfort and relatively low treatment costs. During treatment, they are supposed to provide stationary anchorage (i.e., remain rigid and resistant to load and momentum) and be easy to remove. The limited possibilities to find enough bone volume with sufficient cortical thickness and inter-radicular distance on the vestibular side of the jaws have made the palatal side and para-medial an attractive alternative. The mini-implants placed para-medially, however, sometimes require an additional laboratory construction. This step complicates and prolongs the clinical procedure and increases the cost compared to mini-implants on the vestibular site. However, those mini-implants have the advantage over traditional palatal implants in that they do not require healing time before loading and surgical removal. Palatal implants comparable with prosthodontic implants recommends loading after an appropriate healing period. Immediate loading has, however, been demonstrated to be successful [21]. The insertion and removable of palatal implants is more invasive because of their dimension and surface character. Lately however, devices have been presented that allows for removal of the palatal implants by an unscrewing technique making a second invasive surgical intervention unnecessary (Hänggi M, results not yet published).
The most invasive surgical procedure is needed for the mini-plates. Both palatal implants and mini-plates have though demonstrated higher success rates compared to mini-implants. The described three kinds of orthodontic implant types can be used depending on the clinical situation (i.e., orthodontic movement) and on the force required (i.e., direction and magnitude). The system of preference is also a matter of training, experience, and availability. The most commonly used systems today are mini-implants used both inter-radicular on the vestibular site and para-medial in the palate.
All three orthodontic implant types can be used for direct anchorage and indirect anchorage. Direct anchorage means pull or push directly to the active unit tooth or teeth to be moved, whereas indirect means that the reactive a group of teeth that are pushed or pulled against are supported and stabilized. Indications could be movements of teeth such as distalization, mesialization, intrusion and extrusion of tooth segments for space closure, uprighting of molars, impacted teeth, transversal expansions of palates, and protraction and retraction of the entire maxilla and mandible (Fig. 12.2).
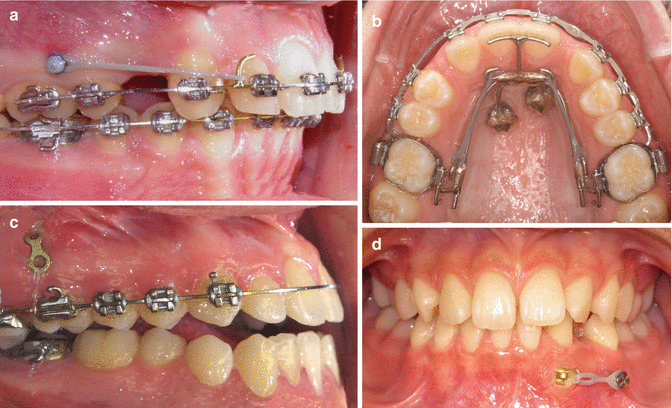
Fig. 12.2
Example of clinical applications. (a) Overbite reduction by distalization using mini-implant anchorage (Courtesy A. Ödman). (b) Space closure in a case with lateral agenesis using mini-implant anchorage. (c) Intrusion in an open bite using mini-plate anchorage (Courtesy M. Möller). (d) Extrusion of impacted canine using mini-implant anchorage
Stability
Success and Failure Rate
The literature has presented results of the success/failure rates (ranging from 0 to 100 %) of orthodontic implants. The variation in success rate depends on many factors including type of orthodontic implant, study design, evaluation time, and criteria for success and failure. These variations also make it hard to compare results from different studies. In individual studies, a success rate of >80 % is the most frequently reported [22–31]. There are four systematic reviews [32–35] and two meta-analyses [36, 37] published on success failure of orthodontic implants so far, where one considers experimentally in vivo studies [33]. Reynders and co-workers and Chen and co-workers in their systematic reviews from 2009 presented a success rate for mini-implants being between 0 and 100 %; however, most rates were above 80 % [35] and between 85 and 100 % [32]. In a systematic review and meta-analysis from the same year, Schätzle and co-workers evaluated failure rates for different orthodontic implant types and presented a double failure rate for mini-implants compared to mini-plates and palatal implants according to the following figures: onplants, 17.2 % (95 % CI: 5.9–35.8 %); palatal implants, 10.5 % (95 % CI: 6.1–18.1 %); mini-implants, 16.4 % (95 % CI: 13.4–20.1 %); and mini-plates, 7.3 % (95 % CI: 5.4–9.9 %) [37]. In a more recent systematic review (2012), Tsui and co-workers also identified success rates: for mini-plates, 91.4–100 %; for palatal implants, 74–93.3 %; for mini-screws, 61–100 %; and for prosthodontic implants, 100 % [34]. In a meta-analysis from 2012, a mini-implant failure rate was found to be 13.5 % (95 % CI: 11.5–15.8) [36]. It could be concluded from the results presented here that orthodontic implants present a higher failure rate compared to prosthodontic implants. The almost 100 % success rate for the prosthodontic implants additionally represents a follow-up period over years, while orthodontic implants operate between 6 and 24 months. The consequence from a failed orthodontic implant, although not as harmful as loss of a prosthodontic tooth, could deteriorate the treatment totally and is inconvenient for both patient and clinician.
Primary and Secondary Stability
The overall stability of an implant depends initially on primary stability and later on secondary stability. Primary stability is the mechanical retention in the bone due to displacement and compression of surrounding tissue, and secondary stability is obtained by osseointegration [38]. A study regarding bone healing presented a critical period around the second to third week when the primary mechanical stability is replaced by the secondary biological stability (i.e., when inflammation and osteoclastic activity decreases, primary stability and new bone have not yet been formed) [39]. Insertion torque, an indicator of rotational resistance, and resonance frequency analysis (RFA) are the most often used evaluation methods to characterize primary stability, whereas removal torque, pullout tests, resonance frequency analysis, and histomorphometry are used to evaluate secondary stability.
A systematic review demonstrated that there is no evidence that associates specific maximum insertion torque levels with higher success rates [40]. This seems reasonable since primary stability alone is not responsible for the long-term success; it is only a prerequisite. This finding is supported in a systematic review that demonstrated that not only proper primary stability but also quality and quantity of loading is of importance [32, 41]. Many parameters are important for stability and success, and it is immensely tested and discussed in the scientific literature. In 1980, Albrektsson and co-workers suggested six factors as prerequisites for osseointegration related to the prosthodontic implants: (1) implant material, (2) implant design, (3) implant finish, (4) status of the bone, (5) surgical technique, and (6) implant loading conditions [42]. These factors could be categorized into three groups and be applied to orthodontic implant conditions: (A) factors related to the patient, (B) factors related to the clinical procedures, and (C) factors related to the implant (Fig. 12.3).
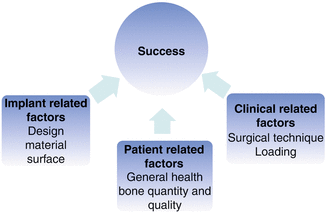
Fig. 12.3
Parameters of importance for orthodontic implant success
Patient-Related Factors
General and Local Health Status
Most important are factors that affect the bone and soft tissues surrounding the implant.
Bone quality and quantity are key factors in creating good primary stability, a precondition for implant success. Numerous studies demonstrate that bone with high density and more cortical bone present better primary stability. These results are supported by in vitro mathematical simulation, in vivo histomorphometrical evaluation, and clinical evaluation using micro-CT and CBCT [43–46]. Factors that affect bone quality and bone quantity include age, gender, location, and health. In some studies, young children have been identified as risk patients with higher probability of failure because they display less bone (i.e., both smaller volume and more immature and less mineralized bone) [47–49]. Moreover, when placing the implants palatally, the midpalatal suture is not ideal for implant insertion because of inadequate mineralization of the interposing connective tissue and the fact that areas of growth do not need to be distressed [50, 51]. However, in a recent meta-analysis, both age and gender have been demonstrated to be of no importance for implant success [36]. Although in vivo studies in general demonstrate higher primary stability for implants in the mandible compared to the maxilla due to more available cortical bone [52, 53], clinically implants in the maxilla have a presented higher success rate than the mandible over time [25, 30, 36, 47]. This differs though from the restorative implants. It might be explained by the increased osteogenic capacity in the maxilla due to the more reactive trabecular bone with better blood supply [38]. Greater stresses created at insertion in denser bone need to be considered as it might affect the success negatively over time [54]. The actual locations within the jaws are important [36], and there are several studies with guidelines of where to install implants optimally in the palatal [55–58] and vestibular [59–63] aspects of the jaws to receive stability and to avoid sinus perforations and root damages (Fig. 12.4). It has been recommended that at least 0.5–2 mm of bone should surround the mini-implant to avoid iatrogenic injury to the root and because close root approximates increase risk of failure [54, 64]. Anchorage from orthodontic implants is of interest not only for the treatment of children and adolescences but also for adults, e.g., as pre-prosthodontic treatment. Bone quality and bone quantity are compromised when a patient becomes old, but could also be a result of diseases or medications. Uncontrolled diabetes and smoking are significant relative contraindications for orthodontic implants as healing following surgical procedures is delayed due to impaired peripheral blood circulation [65]. Medications such as corticosteroids and long-term use of systemic bisphosphonates used for treatment of osteoporosis and certain forms of breast cancer may compromise healing [66].
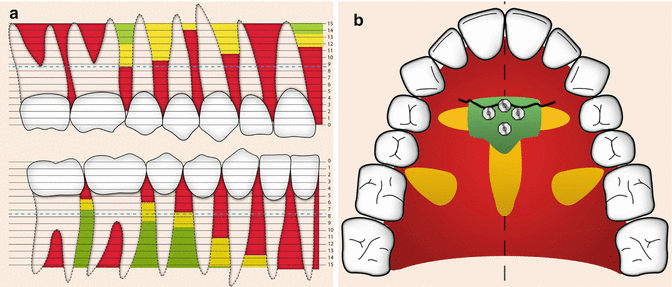
Fig. 12.4
(a) Green areas represent recommended places in the vestibular area to place mini-implants (Courtesy B. Ludwig). (b) Green areas represent recommended places in the palatal area (third palatal rugae) to place mini-implants (Courtesy B. Ludwig)
The recommendations regarding soft tissue placement is to stay within the attached gingiva and out of the nonkeratinized mucosa facial [67] and in the palate when deviating from the midpalatal region not too far posteriorly [68]. Poor oral hygiene and inflammation of the tissue around the implants have been presented as a parameter for failure [30]; however, no specific pathogen has been associated with failure [69].
Factors Related to the Clinical Procedures
Surgical Technique
The basis for success is proper surgical technique (i.e., minimal damage during surgical installation, irrigation to prevent overheating, preservation of the periosteal tissue, and sterile working procedures). The “surgeon’s experience” has been demonstrated to be a cornerstone for implant success [70] where stable installation without substantial wobbling is required [71]. All mini-implants are self-taping; as they turn, they create their own threads. Some of the mini-implants are additionally provided with a sharp cutting tip giving them the potential to be self-drilling. Usually, a self-drilling implant requires no predrilling. However, compared to non-self-drilling implants, experimental self-drilling implants can cause greater bone damage such as pressure necrosis and crack/ruptures in the bone [72]. To avoid these problems, predrilling has been recommended for implants with a larger diameter (≥1.8 mm) and additionally when the cortex is thicker than 2 mm. Predrilling decreases stresses in the bone and prevents fractures of the mini-implant [73–75]. When predrilling is needed, burs with diameters around 0.4–0.6 mm smaller than the actual implant diameter are recommended not to compromise the primary stability [44, 75]. Predrilling depths also have to be considered because maximal insertion torque decreases with predrilling depths [76]. Some studies demonstrate an overall higher clinical success rate with self-drilling mini-implants compared to implants that need a predrilled hole [77]. In vivo experiments confirm that drill-free mini-implants increases primary stability by insertion torque [78] and BIC values [79]. In a recent meta-analysis, however, the importance of predrilling for long-term survival of the mini-implants seemed to be of no significance [36].
The implants can be inserted either manually or with a mechanically (handpiece). Some clinicians prefer to place implants manually because of the tactile feedback such an approach provides; others believe that using a handpiece decreases the possibility of wobbling. Motor-driven insertion also guarantees a constant insertion speed. Because of the potential of the titanium-based implants to osseointegrate, battery-operated driver units with set torque limitation in both insertion and reverse mode have been suggested. Placement torques between 5 and 10 Ncm have been reported to be favorable [80]. However, precaution with battery-operated driver units with torque limitation devices is needed since the accuracy differs between the devices [81]. With respect to insertion depths and implant tightening, low values are insufficient for establishing primary stability as high values could generate excessive high stress and degeneration of interfacial bone and so-called relaxation. In addition, overtightening gives rise to enhanced microstructural damage of the bone [82]. The implant requires at least 1 mm of cortical thickness to avoid failure [83]. Available cortical bone thickness is often not more than 1 mm, so a change in the insertion angle has been suggested and results demonstrate an increase stability by means of bone-to-implant contact [84, 85] and insertion torque and pullout values [86]. A proper angulation might also prevent root damage and failures related to root contact [87]. If the mini-implant fails, there is a possibility to reinstall the implants, and results presented demonstrate that there is no significant difference in success rate for these implants [28].
Loading
The literature demonstrates that proper quality and quantity of loading is important for success [32]. The implant head is connected to and loaded either directly to the active unit through an elastic coil, chain, or springs unit or indirectly to the reactive unit through a rigid wire or casted construction. Indirect loading has been demonstrated experimentally to be more favorable than direct loading [88]. The duration of force application varies and depends on time required to perform the desired tooth movements. Studies present duration of loading time ranging from 3 to 37 months [35]. This survival rate might depend on whether or not the implants have had the possibility to osseointegrate as osseointegrated implants have higher potential to withstand load over time. The force magnitude is also important, and an increased load presents an increased risk of displacement and failure [89]. When the load is within physiologic limits (50–200 g), no correlation exists between the magnitude of force and effect on periodontal parameters [90]. Some studies demonstrated that the direction of the force (CV, CCV) is important [91], but others claim the force direction is not significant [92]. Furthermore, intermittent forces seem to do better than continuous forces in vivo [93].
Immediate loading is a topic of great interest. According to the Cochrane Review Group, early loading is defined as the initiation of implant loading between 1 week and 2 months after surgical insertion, whereas immediate loading is initiated within 1 week after surgical insertion. Others define immediate loading within 48 h or direct after implant placement. Over the last few decades, the proposed healing time before loading has been gradually decreased, but there are groups that still advocate a healing period of up to 3 months [68, 94, 95]. The perquisite for immediate loading is good primary stability. In 2007, a systematic review presented loading in experimental studies. At the time, only two studies were included that loaded the implants immediately. The success rate was higher for the implants that received a healing period compared to immediately loaded implants [33]. Since then, several experimental studies on immediate and early loading demonstrate no negative effect on the bone-healing pattern [53, 96–104]. Ohashi and co-workers in 2006 performed a systematic review on loading protocols for prosthodontic and palatal implants and mini-implants [105]. For the mini-implants, the healing time varied between 2 and 12 months (average of 4–6 months), and the mini-implants were loaded immediately or after 2 weeks. The prosthodontic implants were 100% successful, while the mini-implants demonstrated a failure rate of approximately 10–15 %. In a meta-analysis from 2012, no significant differences of the failure rates of mini-implants could be observed concerning the time of orthodontic force application (i.e., immediate loading (up to 2 weeks) or late loading (later than 2 weeks)) [36].
Although some studies claim increased osseointegration, no study so far has presented convincing evidence of increased early bone healing stimulated by load. This should not to be mixed with adaption mechanisms to physiological loading during remodeling [106, 107]. Parallels could be drawn to fracture healing where the role of mechanical stimuli and strain during the initial callus formation remains unclear. Experimental data has shown that maximal possible rigidity at the fracture site is advantageous until a mineralized callus is formed. After a mineralized callus is formed and the remodeling phase has started, mechanical strains could influence the remodeling and modeling phases of bone healing. The link between mechanical input and remodeling process is historically known as Wolff’s law of bone. Why is it then that immediately loaded orthodontic implants demonstrate similar response as implants with delayed healing? Implant healing is sensitive to micromotion, and motion of less than 100 μm can cause tissue capsulation and failure. Because micromovement might be more harmful than load during the early phase [108], immediate and early loading techniques with light initial force can be applied at an early stage. Loading with a light force may not stimulate early healing, but it could prevent hazardous micromovements of surrounding tissues. This strategy could be compared with the specific guidelines for direct loaded prosthodontic implants that recommend initial splinting until osseointegration occurs to prevent micromotions and failure of immediately loaded implants [109]. Furthermore, it is recommended that if it is possible to have more than one implant in a unit.
Implant-Related Factors
Design (Dimensions and Form)
The lengths of mini-implants vary considerably, and manufacturers offer implant lengths from 4 to 15 mm. In vivo mechanical tests have demonstrated that longer mini-implants provide better primary stability [43, 110]. A too long mini-implant, however, may cause iatrogenic damage including root injury and sinus perforation [43]. Furthermore, increasing lengths decreases mechanical strength [111]. A longer mini-implant must be compensated by a greater diameter to withstand the equivalent mechanical requirements especially during insertion. An oblique insertion angle could be used to enhance contact between implant and cortical plate [84, 85]. Optimal lengths of the implant has been suggested to be approximately 8 mm, and lengths between 6–10 mm are the most commonly used lengths [112]. The length of the implant needs to be determined considering the bone available and the transmucosal thickness, screw angulation, and adjacent vital structures. Moreover, the part of the orthodontic and mini-implants inside the bone should be equal or longer as the part out of the bone.
The mini-implant diameter normally varies between 1.2 and 2.3 mm and is larger for palatal implants. Which diameter to choose is a compromise between the inter-radicular space available and mechanical strengths of the mini-implant. An implant with a smaller diameter (<1.5 mm) may bend or cause breakage of the implants [113]. A larger diameter (>1.7 mm) offers good mechanical stability, but there is not enough space between the roots. As reported before, studies recommend a large variation in the amount of bone required around an implant from 0.5 to 2 mm to be safe and stable [114]. For example, a mini- implant with a diameter of 1.6 mm requires as minimum a root distance from 2.6 mm over the whole length. This limits the potential places for inter-radicular placement to a small number [63]. Root contact during insertion can increase the failure rates compared with mini-screw implants without contact [29, 36, 115, 116]. Tooth position and mini-implant position changes during treatment also need to be considered. Although the mini-implants have proven to be perfectly stable, they are not perfectly stationary if loaded. Studies demonstrate displacement even with light forces [102, 103, 114, 117, 118]. A recent meta-analysis though demonstrated a displacement of 2.3 mm less than for conventional anchorage [119]. Implants smaller than 1.5 mm should be avoided because of the fracture risk especially in the thick cortical bone of the mandible [113, 120]. One study demonstrated that all mini-implants with a diameter less than 1 mm were lost prematurely [27]. This could be explained by the fact that the force required to remove the implant (removal torque) is directly proportional to the square of the implant diameter [79]. However, a larger diameter results in greater micro-damage [121]. Optimal diameters have been suggested to be 1.5–1.7; in vivo studies have demonstrated an increased insertion torque and primary stability with larger mini-implant diameters [45, 110, 121–123]. Some clinical studies demonstrate correlation between success rate and larger mini-implant diameter [25–27, 47], while others do not [29–31]. In a recent meta-analysis, both lengths and diameters have been demonstrated to be of no importance for mini-implant success [36].
Orthodontic implants are either a tapered/conical or a cylindrical shape. Tapered/conical implants have presented higher insertion torque than cylindrical implants [123, 124], and vice versa when tested by means of pull out tests [78, 122, 125]. With an increasing angle of insertion, pullout tests fail to demonstrate differences between the two designs [78]. Similar results have been demonstrated if predrilling is used in conjunction with the tapered implants [76]. Conical implants have a smaller diameter at the apex, which is beneficial in small inter-radicular spaces as they minimize root contact. Although from mechanical aspects, a thinner screw is a disadvantage. Cylindrical implants offer a more even stress distribution, and this helps prevent fracture during placement and removal.
The thread on the implant is the helical ridge, wrapped around the cylinder. The thread varies in design with respect to pitch, flute, and the angle of thread. Flutes are recessed areas in the screw’s cross-sectional area, and pitch is the distance between the threads and angle and the angle of the threads in relation to the long axis. Increasing the dimensions of thread depths and decreasing thread pitch and flute will enhance primary stability in compromised bone; however, increased flutes and deeper threads create higher stresses and increased fracture risk [122, 126, 127]. A symmetric thread form has been proven to be better than an asymmetric thread form [120]. There are five head designs: hook, ball, hole, simple slot, and cross-slot and combinations (Fig. 12.1).
Conclusion
Studies give contradictory results on optimal patient-related factors, clinical procedures, and implant design. In most studies, there are scatters of parameters evaluated at the same time, making it impossible to draw any evidence-based scientific conclusion. Studies need to be designed to provide proper information on independent variables. It might be that the relative importance of a single parameter is of no significance clinically; however, to determine this and to optimize implant design and clinical procedures, all other parameters need to be controlled. If all parameters are optimized independently, the synergetic effect might contribute to an increased clinical success rate.
Material
The first screws and wires used for the purpose of orthodontic anchorage were made of Vitallium and stainless steel [1]. They failed within 1 month and thereafter not much was presented regarding orthodontic bone anchors until three decades later. The first implant made of titanium used for orthodontic anchorage was the mandibular blade implants introduced by Linkow in the early 1970s. This treatment modality, however, never became a widespread treatment modality. Well into the 1980s, and after the invention of titanium, Vitallium implants were still used [3, 6]. Other materials presented were aluminum oxide implants [5] and vitreous carbon dental implants [4]. Also biodegradable materials including biodegradable polylactide acid have been anecdotally tested [128]. In the 1980s, Roberts and co-workers were among the first to use the prosthodontic restorative screw-shaped cp titanium implants [7]. Implants tailored for orthodontics use were made of either cp titanium or stainless steel when they were launched in the 1990s. Most implants today are made from titanium alloys because of its superior mechanical properties compared to cp titanium. Analysis of cp titanium has revealed that removal torque values were dangerously approaching yield stress values because of the small diameter used for mini-implants [129]. Today only one company markets mini-implants made of stainless steel.
The actual osseointegration of the mini-implants is controversial. Most researchers and clinicians categorize mini-implants as being “non-osseointegrated” devices that mainly rely on the mechanical interlocking established at installation (i.e., the primary stability) [130–133]. For mini-implants made of titanium alloy, these statements are questionable because titanium and titanium alloy implants by nature osseointegrate for some months during favorable conditions because of their biocompatibility properties, which have been known for several decades. This well-known fact dates back to the 1950s when Brånemark and co-workers by coincidence discovered that the titanium chambers used for studies of blood circulation in rabbits became permanently incorporated in the bone [134]. The concept of osseointegration was first described by Brånemark and co-workers [135] and originally defined with respect to histological criteria [42]. This definition was criticized for not defining the level of resolution and the amount of bone required to be in contact with the implant. A more recent definition is of a more clinical viewpoint and includes the biomechanical aspects of the implant: “A process whereby clinically asymptomatic rigid fixation of alloplastic materials is achieved, and maintained, in bone during functional loading” [136]. Before these findings, the idea was that only ceramic not metal could be in close contact with the bone and that capsule of connective tissue was developed around all metal implants placed in the body. Thereby the material used in the implant regulated the actual thickness of the capsule and the closeness of the bone to the implant. The material would thereby determine the amount of bone in contact with the implant and stability when the other parameters were controlled [137, 138].
There has been discussion regarding whether metals other than titanium – including Vitallium, tantalum, and stainless steel could osseointegrate. However, titanium differs from these metals by expressing a layer of oxide on its surface. This 100-Å-thick layer of oxide that forms on the surface in contact with air, mimicking ceramics, prevents the metal compounds from directly contacting the bone. Many studies of mini-implants also demonstrate osseointegration [102, 104, 139–141], whereas studies on Vitallium implants appeared to be completely enveloped by a capsule of fibrous connective tissue that varied in thickness [3]. A connective tissue capsule surrounding other implantable materials such as stainless steel does not rule out a functioning implant for limited periods. Bone-to-implant contact values (BIC), representing the magnitude of osseointegration, have been presented as low as 2 and 5 % with the implant still being stable [53, 97]. In addition, implants in dead avascular bone can withstand a load for long periods. Orthodontic stainless steel implants have demonstrated similar success, and bone contact in vivo as titanium alloy mini-implants after a short follow-up [142]. In vitro analysis of these implants suggests that some cells, the osteoblasts, are affected, while others, the fibroblasts, are not [143]. Furthermore, the detected concentrations of ions released have not reached toxic levels in in vivo experiments [129]. It could, however, be concluded that a fibrous capsule is more often expressed when using other materials than cp titanium because they are less corrosion resistant [144]. This results in an increased risk of inadequate long-term resistance of the peri-implant tissues due to mechanical, chemical, and microbial trauma.
The optimal scenario for implants is osseointegration that withstands orthodontic load and at the same time can be removed at the end of treatment with minimal trauma. Some studies claim that the osseointegration is a disadvantage and undesirable because of the risk of not being able to remove it after use [132–145]. Other studies, however, have demonstrated that even surface-modified cp titanium [141] and titanium alloy implants with bone-to-implant contact (BIC) of 75 % [139] could be removed safely. Some studies discuss this possibility to remove the implant as being due to partial osseointegration [139]. The term “partial” osseointegration is confusing and could be questioned since the implants at time of evaluation were functionally stable and demonstrated high values of bone-to-implant contact. Not even successful long-term prosthodontic implants present a 100 % BIC but rather a mean BIC around 75 % with a little higher values for the mandible than the maxilla [146]. “Full” osseointegration is probably disrupted and turned “no” osseointegration by the overload situation created when a force is applied to remove the implant. Secondary failure of osseointegration has been demonstrated due to overload [42]. The simplicity of removing the mini-implants is probably due to the small diameter. Studies have demonstrated that the stability, measured by means of removal torque values, is inversely correlated to the diameter of the implant [147].
Conclusion
If orthodontic implants are made of titanium and titanium alloy, they have the potential to osseointegrate during favorable conditions. Osseointegration is advantageous for orthodontic implant stability and success rate, and it has though been demonstrated that they can be unscrewed without risks.
Implant Surface
The first Brånemark titanium implants launched had a turned comparatively smooth surface. However, since osseointegration depends on biomechanical bonding (i.e., ingrowth of bone into small irregularities of the implant), the topography and especially the roughness of the implants were areas of interest and the subject of numerous studies. Guidelines have been presented regarding how to perform and present parameters of topography in a standardized way to allow for comparisons [148]. Based on experimental evidence from the mid-1990s, a surface roughness of about 1.5-μm Sa (the average deviation in height from a mean plane) and Sdr (surface enlargement due to surface topography as compared with a flat reference area) of 50 % was defined as optimal for osseointegration [149]. This was rougher than the original: the turned Brånemark implant has a surface roughness (Sa) of about 0.9 μm and Sdr of 35 %. Surface orientation has been demonstrated to be of secondary importance for implant-bone integration compared to roughness [150]. A modified surface roughness results in altered protein absorption and subsequent inflammatory process cellular responses in vitro [151, 152]. There are several methods by which the titanium surface roughness can be modified to increase the surface area: physical (blasting), chemical (acid and alkali etching and electrochemical – electropolishing anodizing), deposition (plasma-spraying and solgel), and biochemical methods (proteins and other biomolecules) (Fig. 12.5). However, modifying the surface roughness will affect not only the topography but also the physical mechanical and chemical properties. Efforts have been made to modify the chemical composition to add a biochemical bonding to the biomechanical bonding. The theoretical benefit of a chemical bond would be earlier attachment because it is hypothesized to occur more rapidly than bony ingrowth. Materials that have the capacity to bond to living tissue are defined as “bioactive,” and Hench and co-workers in the 1970s described the first bioactive material, bio-glass [153]. Jarcho and co-workers were the first to present indications of a possible direct bone bonding to hydroxyapatite (HA) [154]. The mechanism suggested was ion exchange resulting in an apatite layer that adsorbed proteins that serve as growth factors for bone cells. The “bioactive” properties of these materials were based on morphological observations of the tissue coalescence by TEM and apatite formation in vitro and in vivo. However, bioactivity or chemical bonding is difficult to prove, and evidence presented is of an indirect nature. Poor mechanical properties of these materials make them unsuitable for load-bearing, clinical applications. To improve these properties, titanium surfaces were coated with calcium phosphates using the plasma-spraying technique. The surfaces showed rapid tissue response initially, but at later stages biodegradation and delaminating of the thick coating were frequently observed [155]. Additionally, the line-of-sight problem made the technique inappropriate to use for the coating of more complex shapes. To avoid these problems, alternative techniques – ultrathin coatings of calcium phosphates in solgels, etching with fluoride-containing acids, alkali-heat treatment, and anodization – have been used to make cp titanium bioactive [156]. Most commercial prosthodontic implants today have been subjected to either of these treatments in addition to the machining. Another possible approach to enhance the bone response is to immobilize organic biomolecules including proteins and pharmaceuticals [157]. There are, however, problems to overcome, and to date this is mostly on an experimental level. Nanotubes and meso-porous surfaces that produce systems for slow release of substances are state of the art.
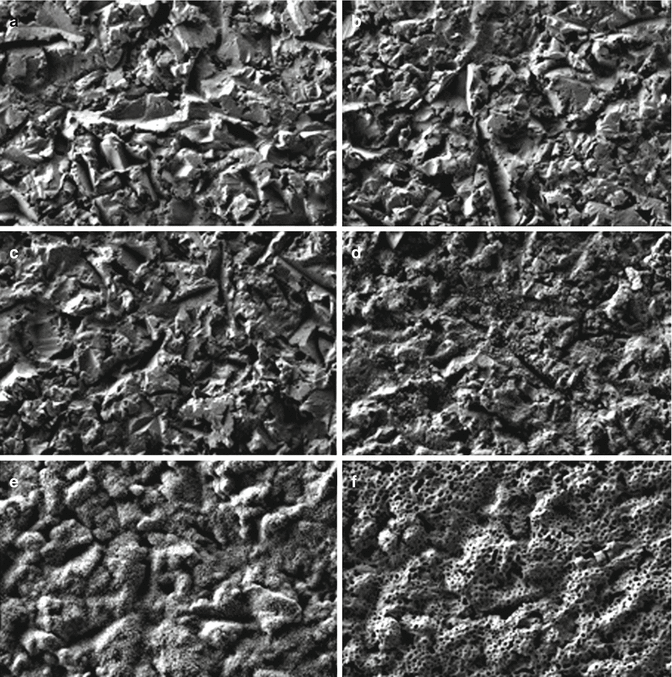
Fig. 12.5
SEM images (×1,000 magnification) demonstrating different surface modifications of titanium implants. (a) Blasted control surface. (b) Blasted surface treated in simulated body fluids (SBF) for 72 h. (c) Nano-hydroxyapatite (HA) surface. (d
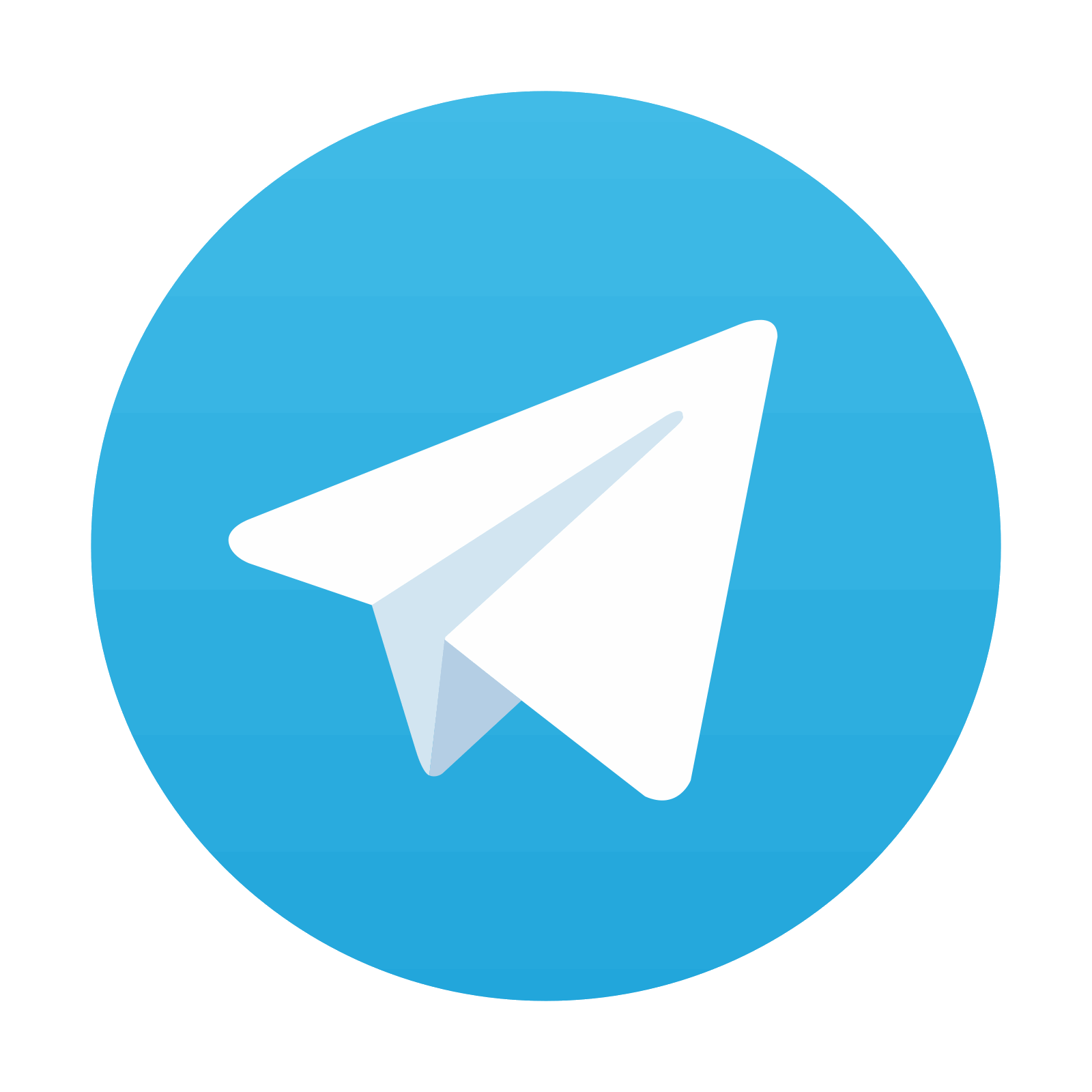
Stay updated, free dental videos. Join our Telegram channel

VIDEdental - Online dental courses
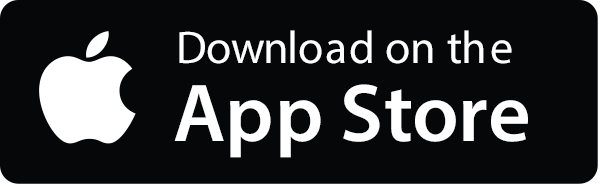
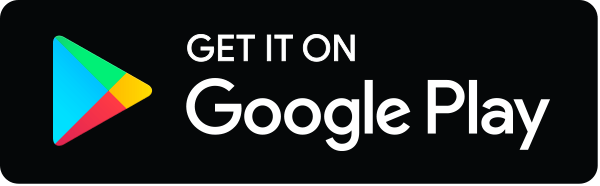