Normal respiratory rates
Age
Breaths/min
Infant (<1 year)
30–60
Toddler (1–3 years)
24–40
Preschooler (4–5 years)
22–34
School age (6–12 years)
18–30
Adolescent (13–18 years)
12–18
Normal heart rates
|
||
---|---|---|
Age
|
Awake
|
Sleeping
|
Newborn – 3 years
|
85–205
|
80–160
|
3 months – 2 years
|
100–190
|
75–160
|
2–10 years
|
60–140
|
60–90
|
>10 years
|
60–100
|
50–90
|
Normal blood pressure of children
|
||||
---|---|---|---|---|
50 % of height
|
||||
Age
|
Girls
|
Boys
|
||
Systolic
|
Diastolic
|
Systolic
|
Diastolic
|
|
1
|
86
|
40
|
85
|
37
|
2
|
88
|
45
|
88
|
42
|
3
|
89
|
49
|
91
|
46
|
4
|
91
|
52
|
93
|
50
|
5
|
93
|
54
|
95
|
53
|
6
|
94
|
56
|
96
|
55
|
7
|
96
|
57
|
97
|
57
|
8
|
98
|
58
|
99
|
59
|
9
|
100
|
59
|
100
|
60
|
10
|
102
|
60
|
102
|
61
|
11
|
103
|
61
|
104
|
61
|
12
|
105
|
62
|
106
|
62
|
13
|
107
|
63
|
108
|
62
|
14
|
109
|
64
|
111
|
63
|
15
|
110
|
65
|
113
|
64
|
16
|
111
|
66
|
116
|
65
|
17
|
111
|
66
|
118
|
67
|
The major systems of interest during a procedure involving oral sedation are respiratory, cardiovascular, central nervous system (CNS), gastrointestinal, and renal. From a monitoring viewpoint, the respiratory, cardiovascular, and CNS are the three main systems of interest potentially affected by sedatives depending on the drug(s) and dosages. For this reason, the clinician will be responsible for closely monitoring ventilation, oxygenation, hemodynamic stability, and CNS functions characteristic of these systems. There are no direct measures typically monitored for the GI and renal system. However, familiarity with their function as it relates to pharmacokinetic and pharmacodynamic management of the sedative agent(s) will be important during clinical procedures and expectations as well as for the process of advisory information and feedback instructions provided by the clinician to the patient or parent.
The act of monitoring can and does, in most cases, involve both a trained professional and assistant, who use clinical observation techniques and various monitoring apparatuses (e.g., precordial stethoscope) according to sedation guidelines [2, 3]. It must be emphasized that reliance only on feedback from monitoring apparatuses during a procedure is not sufficient in promoting optimal patient safety. For instance, a clinician who simply places an oxisensor from a pulse oximeter on the patient knowing the monitor may sound an alarm if oxygen desaturation drops to a certain level and otherwise focuses on the intricacies of the operative procedure is inadequately monitoring the patient (especially if the alarm function has been silenced!).
Monitoring can be both invasive and noninvasive [4, 5]. However, noninvasive monitoring is used for oral sedation involving children because of its convenience, lack of painful stimulation, and usual acceptance by the child once the monitoring is explained or shown to the child in an appropriate fashion.
Monitoring apparatuses can further be divided into non-electronic and electronic monitors (see Table 7.2). Non-electronic monitoring is simple in concept but requires direct clinician-to-patient interaction in order to obtain a measurement (e.g., obtaining respiratory rate with a stethoscope). Automated electronic monitors provide a display of the measures being monitored. They may also provide a printed output that is date-time stamped. The design and sophistication of electronic monitors have increased dramatically since the introduction of microprocessor chips in the 1970s and 1980s. Today, the monitors are relatively small in size and often contain multiple measured parameters within a single unit. There are many types of monitors on the market [3]. In dentistry, the pulse oximeter was recently found to be the most frequently used monitor, although others are used as well [6].
Table 7.2
Summary of monitors used frequently in sedating pediatric dental patients
Monitor
|
Measurement
|
Critical factors affecting signal
|
Advantages
|
Disadvantages
|
---|---|---|---|---|
Pulse oximeter
|
Absorption and ration of red an infrared light representing degree of hemoglobin saturation. Also determines heart rate via plethysmography
|
1. Sensors must be directly opposite each other
2. Patient movement
3. Crying, sobbing, and Valsalva maneuver
4. Pressure on vascular vessels above probe (e.g., blood pressure cuff)
5. Cold limbs
|
1. Safe and noninvasive
2. Simple to use
3. Information rapidly available for clinical decisions
4. Indirectly and secondarily indicates respiratory exchange
|
1. Non-reusable probes are expensive
2. Finger probes can be easily dislodged by uncooperative patient. Toes work well with probe taped (gently) in place
3. Emitted light source may cause burning sensation
4. Does not directly determine airway patency
|
Automated blood pressure cuff
|
Simultaneously records oscillations and bladder pressure. Also, determines heart rate via plethysmography
|
1. Cuff size must be appropriate for arm. Too narrow falsely increases absolute parameters; too broad falsely decreases absolute parameters
|
1. Safe and noninvasive
2. Simple to use
3. Determination time usually less than 30 s
|
1. Determination time increased with moving uncooperative patient eventually causing discomfort and potential limb paresthesia
2. Because of #1, may decrease patient cooperation
|
Capnography
|
Expired carbon dioxide
|
1. Nasal probe must not be blocked by mucus or physical barrier (e.g., nasal septum or alae)
|
1. Safe
2. Simple to use
3. Information rapidly available for clinical decisions
4. Indirectly indicates respiratory exchange
5. Directly determines airway patency
|
1. Temporary block of sample line by mucus
2. May register low CO2 values when child is crying or probe becomes dislodged for nasal opening
|
Precordial stethoscope
|
Sounds of heart and lungs/airway
|
1. Placement of the bell on the chest wall (best location for determining airway patency with faint heart sounds is over presternal notch below thyroid cartilage)
2. Extraneous sounds (e.g., noise from handpiece)
3. Fixation to chest wall
|
1. Inexpensive
2. Simple to use
3. Noninvasive
4. Durable
|
1. Picks up interfering vibratory sounds (e.g., from handpiece)
2. Does not determine degree of airway patency
3. If improperly placed, decreases usefulness in auscultating sounds
|
Pulse Oximetry
Pulse oximetry is an indirect means of noninvasively measuring the percent of oxygen saturation of hemoglobin molecules in red blood cells (RBCs) on a continual basis. The percent oxygen saturation of hemoglobin is important during sedation because it gives an indication of how much oxygen is being delivered to metabolically active tissue, especially the brain which requires lots of oxygen to function. Hemoglobin molecules in RBCs become saturated with oxygen (4 oxygen atoms per heme protein) as they pass in the capillary beds surrounding the lungs. The saturated hemoglobin in the RBCs is then pumped throughout the body via the circulation system to metabolically active areas of the body. As the pCO2 in areas of metabolically active tissue rises, it causes a shift in the tenacity at which hemoglobin binds oxygen resulting in a release of oxygen molecules in the area and a desaturation of the hemoglobin. The RBCs containing the desaturated hemoglobin make their way back to the lungs via the circulatory system to be saturated once again.
If there are any conditions or situations at any step along this saturation-desaturation of hemoglobin process, then clinical problems can arise. For instance, and as it relates to sedation, if the upper airway is blocked and not corrected, oxygen cannot get into the lungs, and the desaturated RBCs passing through the oxygen-depleted lungs cannot pick up oxygen to deliver to already deoxygenated tissues rapidly causing death. In this respect, pulse oximetry is a way to indirectly determine if a person is being ventilated and obtaining sufficient oxygen for active tissues. Pulse oximetry does not directly measure ventilatory function which in simplistic terms is the movement of air into and out of the lungs.
The functional mechanism of the pulse oximeter monitor can be described as follows. Pulse oximetry is based on the red and infrared light absorption characteristics of oxygenated and deoxygenated hemoglobin. The wavelength of red and infrared light is 660 and 940 nm, respectively. The states of oxygenated and deoxygenated hemoglobin absorb red and infrared light differently. Oxygenated blood absorbs more infrared light and deoxygenated hemoglobin absorbs more red. Conceptually and mnemonically, one could imagine oxygenated blood as appearing bright red with more red light passing through it so we can “see” that it is red (infrared is differentially absorbed). Conversely, deoxygenated blood appears darker (not as bright) and the red light is differentially absorbed.
The pulse oximeter is a monitor that contains a microprocessor and uses a “lead” or oxisensor that affixes to the patient’s anatomy (Fig. 7.1). The oxisensor contains two elements: a light-emitting diode (LED) and a photosensitive diode. The LED is capable of emitting red and infrared light at a relative high frequency (i.e., 30 per second). Since the eye does not detect infrared light and cannot discriminate frequencies higher than 20 cycles per second, the LED when lit simply appears as a small red light. The photosensitive diode determines the ratio of the two light waves transmitted and not absorbed. When a tissue bed is placed between the two diodes, the ratio of the two light wavelengths that are not absorbed by the tissue can be measured.
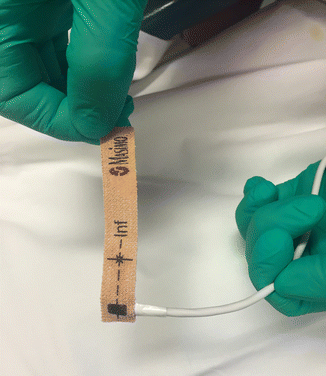
Fig. 7.1
Band-Aid style of oxisensor for pulse oximeter
The dimension of the tissue bed changes ever so slightly when an arterial pulse passes through a tissue bed. Because of the dimensional change of the tissue due to the pulse pressure, the transmitted ratio of light travels over a slightly longer distance per unit time. The microchip processor within the pulse oximeter captures this information, generates, and displays the “signal” as both the heart rate obtained by plethysmography (i.e., change in volume in a tissue bed per unit of time) and the percent of oxygen saturation based on the ratio of the two light wavelengths detected. Thus, the pulse oximeter gives a noninvasive reading of peripheral arterial saturation.
Pulse oximeters not only display information on heart rate and oxygen saturation, they are capable of providing visual and auditory signals as well. Thus, a nice feature of the pulse oximeters is that the audible pitch of each arterial pulse varies with the percent saturation of the hemoglobin. A relatively high pitched sound is heard when the monitor indicates the patient is 100 % saturated (actually, they are slightly less than 100 % saturated at sea level in a normal, healthy adult, but the manufacturers of the pulse oximeter set this value at 100 %). As a patient begins to desaturate, the pitch of the audible sound begins to decrease. In this way, the clinician can not only see what the saturation value is, but does not even have to look up from the patient to know that the saturation level is changing. Also, the frequency rate of the auditory sound indicates whether the heart rate is increasing or decreasing.
Another nice feature of the pulse oximeters is that alarms can be set to sound if the heart rate and/or saturation increases above or below preset values. For instance, most pulse oximeters have a preset heart rate value of 140 and 60 beats per minute for the high and low heart rate thresholds, respectively. Furthermore, for some pulse oximeters, the clinician can reset the high- and low-heart-rate alarm setting at any time when the monitor is turned on and functioning. The same is true for oxygen saturation limits. It is advisable to select oximeters with these features when thinking of purchasing a pulse oximeter.
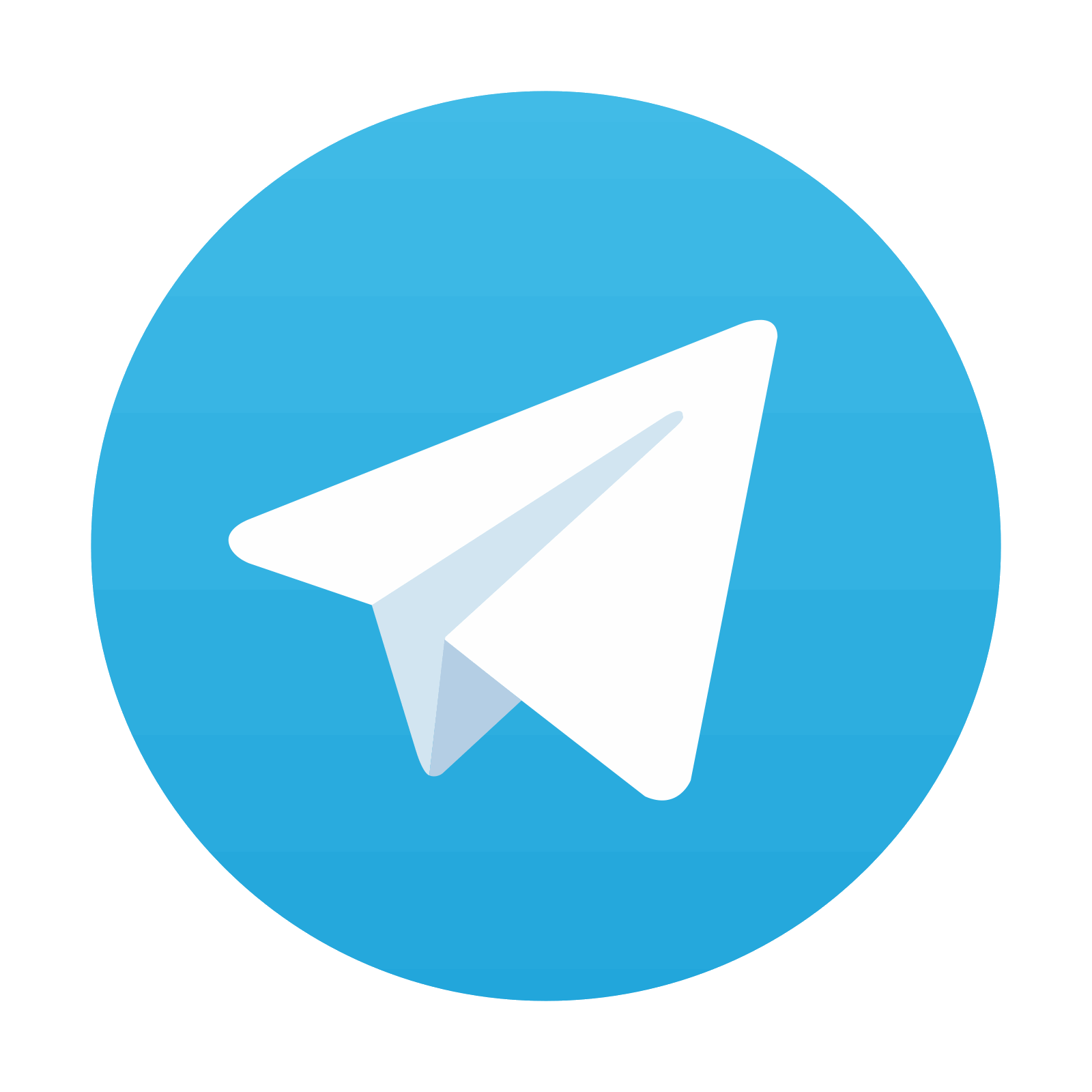
Stay updated, free dental videos. Join our Telegram channel

VIDEdental - Online dental courses
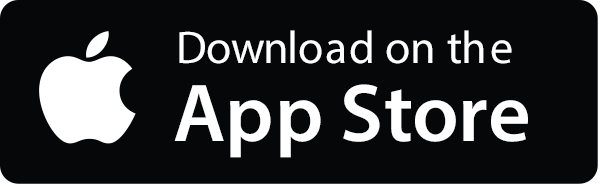
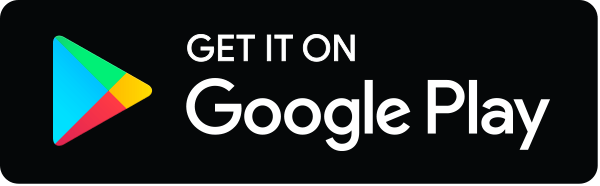