© Springer-Verlag Berlin Heidelberg 2009
Margaritis Z. PimenidisThe Neurobiology of Orthodontics10.1007/978-3-642-00396-7_4
4. Looking Into the “Black Box”
(1)
Marathonos Street 22, 152 33 Halandri, Athens, Greece
4.1 Introduction
It has been traditional to view oral motor behavior in terms of a “black box” a term adapted from the physical sciences to designate areas of a system in which it is not known exactly what is taking place. This view describes input and output with little attention paid to what goes on inside the brain. The black box approach of oral motor behavior handles internal processes by simply ignoring them. The brain that regulates the oral sensorimotor behavior, such as speech, chewing, swallowing, secretion of salivary glands, etc, is traditionally viewed as an impenetrable black box receiving inputs (stimuli) and emitting outputs (responses) with little attention paid to what goes inside the brain. Without information about the intervening central mechanisms most early oral motor behavior research contributed little toward the understanding of normal and dysfunctional motor behavior.
I contend that oral motor behaviors (chewing, speech) are different from the motor behaviors performed in other body segments (walking, writing) in daily life, and orthodontics has much to gain by examining the current state of the art in the motor control and learning area. In this chapter an attempt is made to explore the most recent neurophysiological research on the brain’s mechanisms that are keys to sensation, perception, learning, memory, and motor behavior functions.
4.2 Decoding the Brain Changes
A growing number of researchers are now focusing their attention on the biophysics of the cytoskeleton of neurons, in order to understand its role in the neurophysiology of sensation and perception. Among the reasons to look beyond the classical models of neurophysiology of perception, is the fact that single-celled organisms, such as the paramecium caudatum, have no neurons or synapses, but still exhibit an apparent awareness of and responsiveness to their environment. Thus, the rudiments of sensation and perception lie someplace other than the complex interactions between neurons and their synapses, although the latter are certain to contribute to heightening of sensory input and processing of information, through their strengthening [33]. Hebb [16] suggested that the brain strengthens the neuron synapses according to how often they are used (stimulated) through experiences, resulting in heightening of sensory input and processing in the cortex, which in turn underlies sensation, perception, learning, memory, and motor control.
The currently most accepted view is that the processing of information from the senses occurs at the level of the microtubules within the internal cytoskeletal filamentous proteins of the neuron, that include microtubules, actin, and intermediate filaments. In many respects microtubules are considered the control center, which determines the shape, structure, growth, and function of the neuron [15, 33, 38].
The microtubules are cylindrical polymers composed of tubulin dimers (protein building blocks) with plus and minus electrical ends (dipole). Both tubulin ends undergo polymerization–depolymerization cycles during information processing. Tubulin protein is found mainly in the cytoplasm of eukaryotic cells and it is especially enriched in the brain tissue [15].
Microtubules are most stable and have been hypothesized to be central in cellular information processing. Whenever cellular organization and intelligence are required, microtubules are present and involved. For example, there is accumulated evidence that microtubules are computationally relevant to neurocognition [56, 103, 104]. Woolf [15] demonstrated that microtubules and microtubule-associated protein-2 (MAP2) are proteolyzed with learning, as exemplified in hippocampal neurons of rats with contextual fear conditioning. Similarly, Cronly-Dillon and Perry [105] have shown that neurons of the visual cortex produce massive amounts of tubulin during the critical period (from the day the eyes open to postnatal day 35). The critical period is the time during which synaptogenesis and visual learning occurs at highest rates. Thus, tubulin protein is implicated in developmental cognitive processes.
Specifically, the Penrose and Hameroff [113] model of information processing suggests that information storage, representing structural and chemical modifications of microtubules, occurs in the subsynaptic zone lying beneath the spinous synapses of dendrites, wherein microtubules determine synaptic properties of spines based on previous synaptic activity stored as memory. These changes form the physical basis of long-term memory storage in the microtubules of the subsynaptic zone. The morphological changes in dendrite spines, as well as the formation of new spines and synapses, are key to structural and chemical neuroplasticity that occurs in the microtubules of the subsynaptic zone of dendrites, following learning of experiences [4–7]. The microtubule changes following learning and memory, also form the cornerstone of the neural correlate of perception or consciousness. This implies that the consequence of changes in the microtubules of the subsynaptic zone, following learning and memory of a new experience, will ensure (induce) the emergence of a similar dynamic change in the perceptual state of the brain depending upon the stimulus, through the “Hebbian neuronal assemblies” [38]. In other words, Hebb [16] postulated that functional units corresponding to particular mental states, for instance perception or consciousness might be formed. The functional units are considered as networks or assemblies of many thousands of neurons that can be ignited by particular inputs, and fire in synchrony, and remain active (firing) for hundreds of milliseconds, following which another related assembly ignites, then another, and so on in a phase sequence. Hebb suggested that if the activity of a neural assembly representing a specific set of content exceeds the activity of all other assemblies in some type of competition, it meets the cost of entering into perception or consciousness. In other words, the particular neuron assembly becomes conscious [38].
Several investigators have hypothesized that consciousness is located in a horizontal layer of interconnected cortical neurons sandwiched between ascending (bottom-up) inputs from the thalamus and basal forebrain, and descending (top-down) executive functions from the prefrontal cortex [38]. Bottom-up inputs convey sensory information for general arousal through the reticular formation, as well as emotional context from basal forebrain inputs [106, 107]. Top-down influences include executive functions. Acting together, bottom-up and top-down activations select a neural assembly—a specific subset of cortical–cortical projections—for attention and consciousness [38]. Thus, consciousness is the output of a process that compares available incoming (bottom-up) information against anticipatory executive (top-down) schemata [38, 108].
It is noted that the basal forebrain neurons receive sensory (input) collateral branches from axons en route to the cerebral cortex. In this manner the basal forebrain neurons obtain a general idea of the overall pattern of incoming inputs and then base their outputs on those assessments. The basal forebrain neurons use neurotransmitters acetylcholine and monoamines (norepinephrine, serotonin, and dopamine) [15]. The cholinergic (acetylcholine) neurons contribute to selective attention, whereas the neurons producing norepinephrine, serotonin or dopamine contribute to general arousal of the brain or vigilance. Cholinergic neurons from the basal forebrain are able to contribute to selective attention to a particular sensory stimulus, to a memory, or to a complex amalgam of both stimulus and memory, because they innervate discrete areas of the cortex of a single modality measuring 1–2 mm2. In contrast, the monoamine neurons innervate the cortex less restrictively, i.e., a single noradrenergic (norepinephrine) fiber may provide inputs to many different types of cortex [15].
Taking into consideration that attention is a deliberate action related to a conscious state of mind, cholinergic and monoaminergic inputs diffusely distributed to cortical modules or to the entire cortex, will sample the overall pattern of input en route to the cortex. These inputs are necessary but not sufficient for attention and arousal. Activation by the neurotransmitters glutamate, acetylcholine and monoamines, as well as the biophysical state of the microtubule network (because microtubules can polymerize and depolymerize, they can search for and activate particular subsynaptic sites) are necessary for full conscious awareness. Consequently, the physical sensation of attention and perception or consciousness are not associated with synaptic activity per se. Instead these sensations are associated with the biophysical state of microtubules that regulates polymerization–depolymerization cycles of their building protein tubulin [15].
Thus, the brain is structured as a consequence of the information stimulus. This takes the form of neural events in space and time that correspond to the spatiotemporal relationships implicit in the stimulus. Areas of the brain are excited and others suppressed [58]. Accordingly, the learning–memory abilities of the brain, as well as, the physical sensation of perception or consciousness are “products” of sensory input–information processing of the brain, depending on the transformations imposed upon the cytoskeleton of neurons, as a consequence of the transformations embodied by the stimulus in the senses [58].
The neurotransmitter glutamate mediates the sensory input to the cerebral cortex, where many of the excitatory presynaptic terminals synapse with the dendrite spines [15]. The number of spines and synapses are more abundant in highly arborized neurons, such as pyramidal neurons, suggesting an augmented excitatory input on the pyramidal neurons, which in turn may mediate motor behavior [3, 15].
Glutamate binds to AMPA, NMDA and kainate, receptors located on the membrane of spines, and opens sodium (Na+) or calcium (Ca2+) ion channels depending on the receptor, so that Na+ or Ca2+ enters the cell and penetrates the subsynaptic zone. The ionic influx activates proteins which initiate polymerization–depolymerization cycles of tubulin protein of microtubules, resulting in changes in the conformational state of tubulin, which is critical for effective transport of proteins along microtubules to synapses for their strengthening in conveying the information input [15]. Thus, tubulin protein, the building protein of microtubules in the subsynaptic zone of dendrites, has a clear involvement in cognitive processes, which suggests that learning experience alters the basic structure of the cytoskeleton of neurons. Information of the learned experience is then stored in microtubules as memory, which might or might not reach perception or consciousness [15, 38, 109, 110]. In this regard Alzheimer’s disease, which is accompanied by deficits in intellect, memory and consciousness, has been linked to microtubule degradation [111].
It has been theorized that the interior regions of the microtubules play the role of electromagnetic cavities. The interaction of these cavities with the tubulin binding protein can lead to the emergence of quantum effects, which play a biologically relevant role in the information processing, storage and retrieval done by the neurons [38].
In this context, the interior milieu of microtubules has been linked to a caged quantum bit (qubit). Conventional computers represent digital information as binary bits of either 1 or 0. Quantum computers can represent quantum information as superpositions of both 1 and 0 known as qubits, and are capable of quantum computation. The conformation states of individual tubulins play the role of bits in computers. The conformation states of tubulin protein then are considered the essential input–output function of the neuron [33].
Accordingly, a large number of authors have provided various arguments for microtubules being pivotal to consciousness. In particular, because they may well form the central nervous system of the cell, since microtubules do propagate signals in cells. It has thus been suggested that if one assumes that the phenomenon of consciousness depends on cellular processes, then nature has provided the necessary structures (microtubules) to operate as the basic substrate of quantum computation, provided there are indeed quantum effects in consciousness [33]. Bohm and Hiley [112] proposed that the wave function in the quantum superpositions (particles/systems existing in multiple states or locations simultaneously, governed by a quantum wave function) contains active information that guides the movement of particles along the microtubules, and that consciousness is associated with this movement and active information. The quantum superimposition state in the microtubules is considered to collapse at the supramolecular level due to interaction with the classical environment or the conscious observer [38].
In this view, the currently most accepted theory of consciousness, the Penrose-Hameroff “Orch-OR” model [38, 113] suggests that our brains are quantum mechanical in their processing of information and that the process of human consciousness cannot be simulated classically. They then proposed that consciousness is a sequence of quantum computations of information in the microtubules within brain neurons, shielded from decoherence to reach the threshold for objective reduction (“OR”). The quantum computations are “orchestrated” (hence, “Orch-OR”) by neural/synaptic inputs and extend throughout cortex by tunneling through window-like gap junctions between neurons. Consciousness emerges in dendrites of cortical neurons interconnected by gap junctions (electrical synapses) forming a giant neuron or “hyperneuron,” in which all neurons fire in synchrony (simultaneously).
Gap junctions or electrical synapses occur between neural dendrites, between axons and axons, between neurons and glia, between glia, and between axons and dendrites —bypassing chemical synapses [114, 115]. Ions, nutrients and strands of DNA or RNA pass through the open gaps, so gap junction-connected neurons have both continuous membrane surfaces and cytoplasm, and the neurons are electrically coupled, depolarize synchronously and behave like one giant neuron (hyperneuron) [116].
Chemical synapses and axonal action potentials convey inputs to, and outputs from, conscious processes elaborated in hyperneurons. The experiential action potential from the peripheral receptors reaches the cortical dendrites and alters the conformation state of tubulin protein of microtubules. The tubulin subunits of microtubules are the fundamental units of information. Computations are then carried out by the tubulin subunits which spread all over the cortex through the gap junctions generating the conscious state of the brain [38, 113].
It has been suggested that preconscious experience or thought exists in terms of multiple quantum states, and the conscious experience is realized when one of the many possible states prevails. This means that the conscious mental events can be assumed to correspond to quantum collapses of superposition states at the level of macroscopic brain activity [38].
The perspective that could be advanced is that the oral senses are “online” with the brain, so their information input and processing is involved in learning and memory storage abilities, at the same time also giving rise to a conscious experience in the form of perception or consciousness. This may occur when a critical level of complexity and interaction is reached between cortical neurons connected by gap junctions. In other words, if the activity of a functional unit (hyperneuron) corresponding to a particular mental state exceeds all other functional units in some type of competition, it meets the cost of entering perception. Hence, perception chooses reality, while most other mental activities, including motor activity are normally nonconscious activities [38].
Gap junction networks (hyperneurons), which may transmit through the gap junction quantum states from neuron to neuron individual proteins or strands of DNA or RNA molecules, have been shown to be widely prevalent in the brain and to mediate synchronous firing of neurons in various frequencies of the electroencephalogram (EEG). Among these the so-called gamma coherent 40-Hz neuronal activity correlates best with consciousness [117–119]. Clinical signs of pain awareness, pupillary size, heart rate, blood pressure, lacrimation, diaphoresis, mucus secretion, EEG power spectrum/40 Hz, etc, are used to indicate adequate anesthesia and lack of consciousness. While consciousness is erased during general anesthesia, nonconscious brain EEG and evoked potentials continue, although they are reduced [38].
The conclusion seem to be that the information processing in the brain and the resulting structural and chemical changes of the brain can describe the contents of the black box. The brain is “online” with the mouth continually receiving and processing messages from the senses. The information input reaches the microtubules of the subsynaptic zone of the dendrites, that give rise to spines, and induces transformations of the protein tubulin. The resulting changes in the brain can be seen as forming a continuum, i.e., a series of complex correlated mental activities, depending upon the stimulus, which underlies somatic sensation-perception, attention and awareness (arousal of the brain), and learning and memory (cognitive functions), as well as motor output models to muscles through the pyramidal and extrapyramidal tracts [15, 38, 58].
During growth and development gap junctions link cortical pyramidal neurons into functional units (hyperneurons) emitting outputs to the motor nuclei of the trigeminal nerve, resulting in oral sensorimotor functions, such as speech and chewing. A single pyramidal neuron has numerous gap junction connections, of which only some are open at any one time, with rapid openings and closings regulated by the microtubules through their information-processing transformations [120]. This suggests that gap junctions are as dynamic and mutable as those crafted by chemical synapses [121]. Thus within each cerebral hemisphere there is no apparent limit to the extent of gap junction networks, which may form a large continuous neuron syncytium that could even extend to both hemispheres [122, 123].
In this view, thalamocortical neurons generating synchronous alpha and theta cortical activity in the EEG, are linked to gap junctions in the thalamus [123], so that thalamocortical projections (or trans-corpus callosum pathways) could join the two cerebral hemispheres in hyperneurons to account for the bilateral synchronous contraction of the masticatory muscles [38]. Accordingly, abnormal coupling of the cerebral hemispheres in hyperneurons might underlie the dissociation of the left and right masticatory muscle activity in the unilateral posterior cross-bite malocclusion of the teeth [124, 125], suggesting that the dissociation of bilateral activity of the masticatory muscles in the unilateral posterior cross-bite malocclusion of the teeth is due to abnormal brain function.
In this context, the diagnosis of sensorimotor dysfunction of malocclusions of the teeth associated with oral facial imbalances through the information-processing approach is a far different skill from the classical approach. This is because it enables the orthodontist to view the patient as an active processor of information and hence mediate between the patient and the dysfunction by inducing brain neuroplasticity through the orthodontic corrective experience. This implies that neuroplasticity of the brain is responsible for the changes in motor behavior seen in malocclusions of the teeth following orthodontic treatment. Indeed, neuroscientists widely accept that cognition and perception are correlated with the physiological function of the brain [33, 85].
Unlike the traditional orthodontic diagnostic approach, which views only the peripheral behavioral products of the central nervous system, the application of the information-processing model to oral sensorimotor behavior focuses a special interest on the neural events that are necessary for giving an oral conscious experience and how it may regulate oral motor behavior. Thus, the information-processing approach stresses a complete new conceptualization of oral functions through the description of the precise neural events in the brain that underlie these functions, which eventually will provide practitioners with a rationale for correcting dysfunction of the mouth. For example, employment of the information-processing approach allows the practitioner to compare the ways in which different aspects of oral behavior are structured in memory, so that common properties can be discussed. Also, the practitioner who understands the processes that underlie motor behavior is aware of the patient’s probable cognitive strategies in situations of discomfort or dysfunction and may contribute to diagnose the source of perceptual-motor deficits. Finally, it is obvious that if orthodontics and dental medicine are to advance, there needs to be a better understanding of the executive centers of the brain, such as the prefrontal cortex, whose output in the form of conscious action focuses on behavior including the oral–facial region.
4.3 Looking into Conscious Brain – the Prefrontal Cortex
The prefrontal cortex seeks continuous sensory stimulation to maintain arousal for attention and awareness. Arousal of the brain guides our thoughts and behavior through strengthening of synapses resulting in enhanced communication and processing of information which in turn induces the conscious state of mind and the control of muscles. Conscious actions and/or consciousness are key to successful life. We need to have clearly defined goals. When we know what we want, we are more likely to change our behavior and the prefrontal cortex function to get it [199].
The prefrontal cortex is the most evolved part of the brain. It occupies the front third of the brain, underneath the forehead. It is often divided into three sections: the dorsal lateral gyrus, the inferior orbital gyrus, and the cingulate gyrus. The dorsal lateral gyrus and the inferior orbital gyrus are often termed the executive control center of the brain dealing with output in the form of action which is involved in the active roles of decision making and “working memory” in a space–time representation of our “sense of future” and of our will or intent. Overall, the prefrontal cortex is the part of the brain that watches, supervises, guides, directs, and focuses the behavior. The prefrontal cortex also helps to solve problems and see ahead of a situation on the basis of experience. This is also the part of the brain that helps in learning from mistakes. People who have trouble learning from experience tend to have poor prefrontal cortex function. They tend to make repetitive mistakes. Their actions then are based not on experience, but rather on the moment, and on their immediate wants and needs.
The prefrontal cortex (especially the dorsolateral prefrontal cortex) is also involved with sustaining attention span. It helps focus on important information, while filtering out less significant thoughts and sensations. Attention span is required for short-term memory and learning. The prefrontal cortex actually sends signals to the limbic system and sensory areas of the brain when you need to focus, and decreases the distracting input from other brain areas [199].
People with attention deficit disorder (ADD) have neurological dysfunction in the prefrontal cortex characterized by a short attention span, which is the hallmark of this disorder. People with ADD have trouble sustaining attention and effort over a prolonged period of time. Distractability, difficulty in learning from past errors, hyperactivity, etc, are some of the problems of ADD. Children with ADD have longstanding problems paying attention in school, and to regular routine everyday matters. When they try to concentrate the activity of their prefrontal cortex decreases, rather than increases, as in normal behavior. When the prefrontal cortex is underactive (neurotransmitter abnormalities may decrease prefrontal cortex activity), as in ADD, too many sensory stimuli bombard the brain and the cerebral cortex is suffering of sensory overload leading to sensory deprivation (see Chap. 3).
Clinical neuroimaging studies of the prefrontal cortex, using for example single photon emission computed tomography (SPECT) which measures cerebral blood flow and metabolic activity patterns, often involve two scans, one in a resting state and a second during a concentration task. In evaluating brain function, it is important to look at a working brain. When the normal brain is challenged by a concentration task, such as mathematical problems or sorting cards, the prefrontal cortex activity increases. In certain brain conditions, such as ADD and schizophrenia, prefrontal cortex activity decreases in response to an intellectual challenge. Integration between the ascending (bottom-up) sensory information from the thalamus and the descending (top-down) executive function of the prefrontal cortex [106, 107] may be affected by neurotransmitter abnormalities [199]. On the other hand, experimental stimulation of the orbital gyrus is known to induce rhythmic opening and closing movements of the jaw resembling masticatory movements [197]. It is then hypothesized that oral neuromuscular dysfunction during chewing includes prefrontal cortex dysfunction through neurotransmitter abnormalities affecting the bottom-up and top-down sensorimotor integration function.
4.4 Programming the Oral Senses for Learning
The effectiveness of an environmental stimulus for processing information in the cerebral cortex is not governed by the volume of the sensory input or the direction of change, but by the amplitude and the rate of change of the stimulus [71]. The sensory receptors respond to the perceptual environment by incorporating ordered and variable environmental stimuli into a personal perceptual scheme. The process consists of extracting useful information from the welter of “noisy” sensations with which the organism or mouth is constantly bombarded [69]. The absence of order (meaningful) or variation in the stimuli tends to degrade the cortical perceptual mechanisms [82]. Perception is always selective and is always testing the sensory modalities that are essential for the maintenance of the scheme of the mouth. With breakdown of this internal frame of reference it becomes increasingly difficult and in fact impossible to structure the environment, and to impose constancy and stability of the perceived natural world. As a result, contours fluctuate, ambiguous figures alternate rapidly, and simple geometric figures change in size and shape [82].
The newborn infant, however, has not yet learned which of the incoming environmental stimuli are meaningful for survival and well-being and which are not [82]. The environment sends information signals in the form of concepts or universals (to use Aristotelian language). If the oral senses are to acquire meaning and become incorporated into the perceptual scheme of the mouth, they must develop models of programming allowing the senses to match to the significance of events that occur in the environment, through learning procedures [69].
In this view, the perceptual organization development of the organism or mouth consists of the capacity to utilize the senses to extract information from the environment in order to build the brain. Without such prior learning of models of the environment, the centrifugal control of afferent sources is without a program, without a basis for predicting that certain events are more likely than others or preclude others (inhibition), and have no basis for selectivity toward stimuli [69].
The prediction of the brain that certain events are more likely to occur than others is based upon several considerations about the nature of perceptual development. The widely accepted view is that continued contact with a rich sensory environment permits the development of differentiation of spheres of activity of sensory modalities, of events within modalities [69]. Early sensory deprivation, prevents such differentiation, and prevents the development of selective gating and construction of models of the environment. It also prevents the development of efficient strategies in motor behavior, since the appropriate responses have not being learned. Behavior development depends on cognitive growth, which consists of learning how to handle the transformations that happen in the brain, following information input [15, 102].
4.5 Oral Learning and Memory of Experiences
The brain changes whenever an animal learns a new behavior, and it is assumed that the change in the brain is responsible for the change in behavior [15]. Once a change has occurred, however, it becomes important to identify the neural substrate that is responsible for the recently acquired adaptive behavior.
The traditional view is that the synapse is the only computationally relevant apparatus in the neuron, i.e., learning occurs in the synapses between the neurons, and the information is stored there as memory [16]. In this view Hebb [16] first proposed that synapses might be reorganized (strengthened) with learning and memory. Hebb suggested that the more a synapse is used, the stronger and more efficient at transferring information it becomes. This enhances neuron communication and information input, which results in learning or enrichment of experiences. Use in turn is driven by experience. Our experiences use specific circuits and synapses, thereby strengthening those very synapses.
Subsequently there have been hundreds of studies examining brain tissue for synaptic reorganization following learning or following comparable physiological stimulation that might resemble learning of neural events. For example, Bliss and Lomo [17] performed experiments in rabbits. They stimulated with a strong repetitive stimulus or tetanus the bundle of nerve fibers entering the hippocampus, a memory center of the brain. The fibers of the bundle form synaptic connections with a special group of target neurons inside the hippocampus. Bliss and Lomo recorded the electrical responses from the target neurons and observed that the shock stimulation magnified significantly the electrical responses from the target neurons, compared to normal responses to a low frequency stimulus. In neurophysiological terms the target responses were potentiated by the test shock stimulus. Bliss and Lomo concluded that increased stimulation for seconds results in dramatic strengthening of synaptic communication, neuron responsiveness, and speed of response that persists for six hours after the increased stimulation has stopped.
Thus, a brief electrical experience lasting some seconds on the presynaptic fibers caused long-lasting responses in the hippocampal memory neurons. The increased stimulation of the neuronal pathway entering the hippocampus resulted in greater synaptic strength, which heightened the function of the pathway in transferring information, as well as accelerated the information processing in the hippocampal neurons, which stored memory of the learned experience. The incorporated memory in the hippocampal neurons allowed them to respond for hours after the stimulus had stopped. In this dynamic view, the memory changes do not consist simply of storage of information. Memory incorporated by experience into the brain alters brain function. Specifically, Bliss and Lomo [17] discovered that in the hippocampus there are special neuronal circuits serving memory, which can show long-term potentiation (LTP). This means that the specialized neurons have the capacity to respond to brief experiences and retain through their memory the firing pattern for long time after the stimulus has stopped [2, 17].
While oral experience increases the strength of synapses, resulting in heightening the flow and processing of information in the brain, on the contrary idleness of the mouth decreases the strength of synapses and impairs learning and memory ability. Accordingly, the LTP has its mirror image, long-term depression (LTD) of oral neural activity [2]. This means that the synapses may undergo graded strengthening or weakening depending on their use (exercise). The brain continually monitors experiences and use of synapses and adjusts accordingly their strength in conveying action potentials. The brain emerges as an ever-growing changing structure that builds its structure and function through learning of experiences [2].
In order to induce LTP, a firing intensity threshold must be reached in the hippocampus memory neurons. This can be done by stimulating a single input neural fiber with a strong stimulus, or many input fibers with a lower intensity stimulus. This implies that weak inputs from many sensory modalities, converging on the same neuron can be bound together in the memory neurons and induce LTP [2]. For example, weak stimulation of the taste fibers and weak stimulation of the visual fibers carrying a representation of an apple could associate the taste and the sight of the apple in memory through LTP of hippocampus neurons.
The molecule that senses that the communicating taste and visual neurons are firing simultaneously is the neurotransmitter glutamate, which is responsible for relaying sensory information throughout the entire central nervous system [15]. Glutamate is an excitatory amino acid released into the synapse in response to an action potential of the transmitting neuron. Glutamate stimulates electrically the signal receiving postsynaptic neuron. Glutamate excites the postsynaptic neuron by binding to special receptors on its surface membrane. The receptor is called NMDA (N-methyl-d-aspartate). NMDA receptor is specialized for inducing LTP and storage of memory in the synapses through their strengthening [2]. Experimental data, however, do not consistently indicate that changes in synapses following learning are stable. Thus, a new paradigm may be needed to replace the long-held notion of Hebb’s [16] view that information storage occurs in the synapses.
The widely accepted new learning and memory model advanced by Penrose and Hameroff [113] suggests that information storage as memory occurs in microtubules of the subsynaptic zone of dendrites that gives rise to dendrite spines and synapses. In this view, microtubules and MAPs have a clear involvement in learning and memory storage of experiences. Microtubules in the subsynaptic zone are capable of storing important memory information concerning where spines were originally located in the dendrites, and to use that information to determine which spinous synapses will persist or reappear in the event of dynamic retraction following learning a new experience [15, 33, 38].
According to the new learning–memory model the binding of glutamate to NMDA receptors on the surface of the signal-receiving postsynaptic neuron will open calcium channels permitting the electrically charged calcium ion (Ca2+) to enter the neuron. Once inside the cell, Ca2+
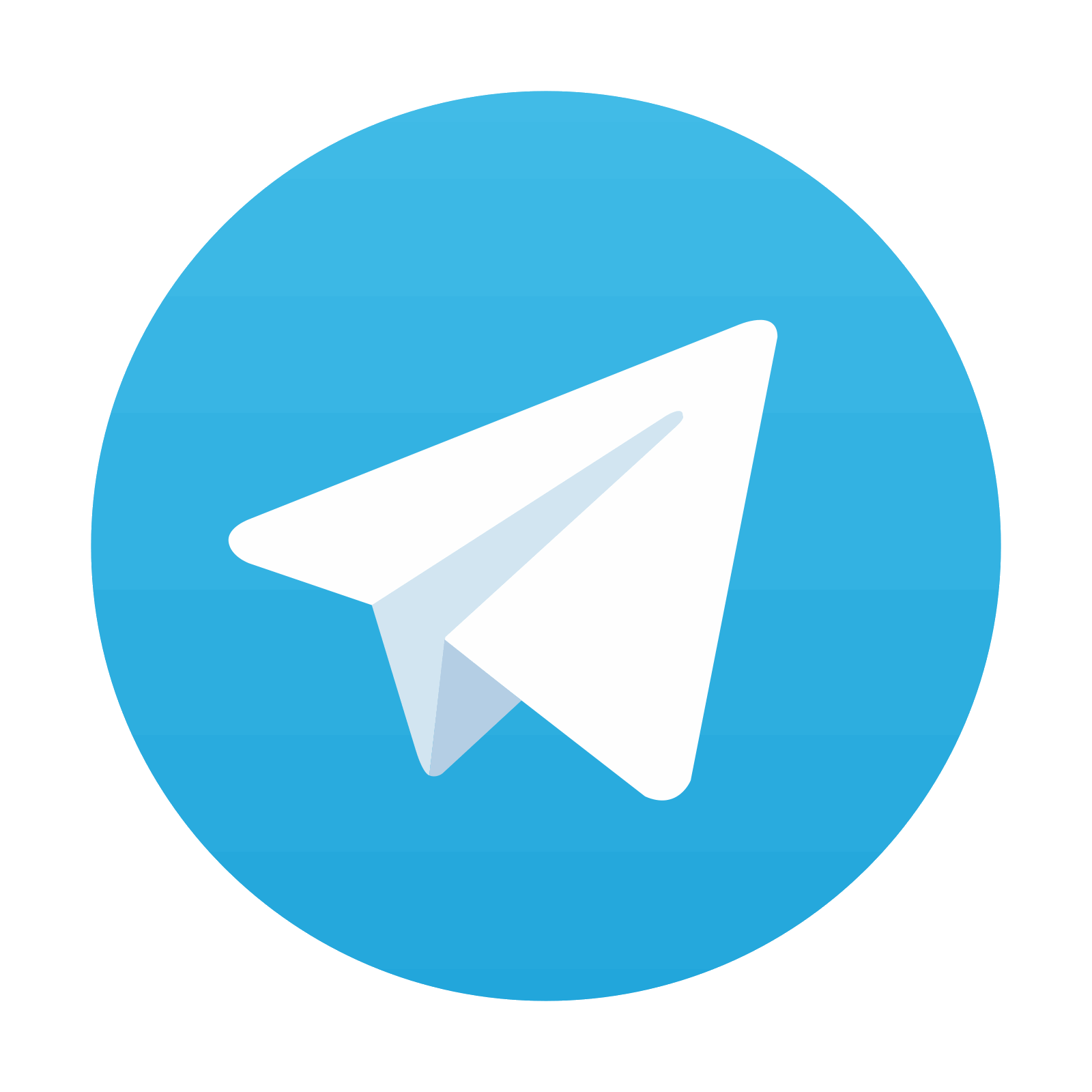
Stay updated, free dental videos. Join our Telegram channel

VIDEdental - Online dental courses
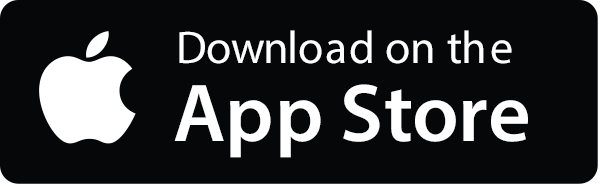
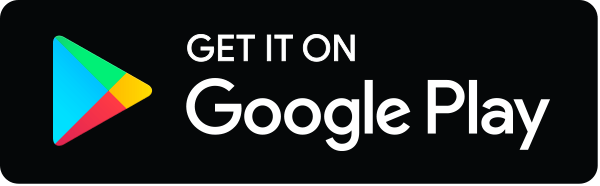