© Springer-Verlag Berlin Heidelberg 2009
Margaritis Z. PimenidisThe Neurobiology of Orthodontics10.1007/978-3-642-00396-7_1
1. Experience Changes the Brain
(1)
Marathonos Street 22, 152 33 Halandri, Athens, Greece
1.1 Introduction
The brain is the seat of feelings and behavior. Your brain creates your world—a radical statement about ordinary thinking. It is your brain that perceives and experiences. Everything begins and ends in the brain. How our brain works determines the very quality of our lives. Our brain patterns help us with our behavior, motor skills, work and being successful in our day-to-day lives. When behavior becomes abnormal, however, often there is something the matter with the patterns in the body’s computer—the brain.
Unfortunately, there are many dental professionals who lack sophisticated information on how the brain actually works. They believe the oral behavior of their patients is primarily the result of environmental stress or conditioning, and do not consider the possibility that it may be based on abnormal brain physiology. I believe we need to understand the role of brain physiology along with other factors such as stress or conditioning before we can design successful oral orthodontic reconstruction treatments for people.
The prevailing concept in orthodontic practice holds that the sensory and motor functions or behavior of the mouth, which is intimately related with the development of the oral–facial structures, is controlled by the brain and, therefore, cannot be influenced by therapeutic measures [1, 206]. This concept is not a flimsy one. It is based on a century-old dogma that mammalian neurons do not divide after development, and they are preprogrammed to develop normally. That is, each infant neuron contains all the information necessary to live, develop, and be well. In other words, the entire plan, the blueprint for a neuron’s life, is in its genes. The brain is a complex but a static, immutable, hard-wired switchboard that cannot be changed [2]. Accordingly, the dogma that the nervous system is static has prevented a meaningful analysis of the interplay between neuromuscular activity and skeletal morphogenesis, through which an understanding of the etiology of malocclusions of the teeth could emerge.
Recently, however, two new discoveries have revolutionized our views. These are: the structural changes of synapses by the firing experience of neurons, and the discovery of growth factors in the brain. These are discussed next.
1.2 Oral Experience Strengthens Neuron Synapses
Neurons are brain cells that are involved in communication of information. The strength of information processing performed by a neural circuit depends on the number of synapses between neurons [3]. For years scientists imagined that the electrical impulses and the chemical signals (neurotransmitters) that neurons use to communicate with each other, do not change the structure of the synapses. They thought that a synapse simply transmits the action potential signal passively, like a telephone, without itself changing [2].
Hebb [16] first proposed that synaptic knobs might be reorganized with learning. Since then there have been hundreds of studies examining brain tissue for synaptic reorganization following learning or following comparable physiological stimulation that might resemble learning-related events.
After years of experimental studies, however, researchers have discovered that the neuron synapses do indeed change with the firing experience of neurons. For example, it has been found that increased electrical stimulation for ten seconds of hippocampal memory neurons results in dramatic strengthening of synaptic communication and increased neuron responsiveness to neurotransmitter signal molecules (increase in the number of receptors projecting through the surface membrane of neurons to which the neurotransmitter is attached), that persists for about six hours after stimulation has stopped. These changes in the memory neurons alter brain function. Specifically the changes in the memory neurons of the hippocampus accelerate information processing through strengthening of the synapses of the neural pathway [17]. In another experiment rats were taught to navigate through a complicated maze while the electrical activity of the hippocampal memory neurons, especially critical for spacial memory, was monitored. As the rats learned to navigate the maze successfully, the hippocampal neurons fired with a characteristic unique pattern which was associated with learning of the particular task [59].
Many studies have provided evidences that chemical and structural modifications of dendrite spine synapses underlie much of the plastic changes in the brain in response to learning an experience [3–5]. Morphological changes of the dendrite spine are key to its plasticity following processing of information [3, 6, 7]. Electrical stimulation of neurons leads to the formation of new dendrite spines [3, 8, 9]. In this regard, dynamic changes of the actin cytoskeleton of the spine have been implicated in the formation of new spines [3, 10, 11]. The actin cytoskeleton of the dendrite spine plays a central role in synapse formation and maintenance [3, 12]. Processing of new information in neurons causes remodeling of actin cytoskeleton protein and modifies synapse stability, which may lead to formation of new synapses [13–15].
Thus, we now know that synaptic change clearly occurs with learning. Synapses are not unchanging hard-wired connections between neurons as previously assumed. Synapses continually strengthen, weaken, grow, and shrink throughout the life of the nervous system in response to experience. A number of new studies have further addressed the question whether these kinds of learning-related plastic changes are permanent, and the consensus is that they are not. The question that arises then is how learning-related information is permanently stored [2, 15]. Several ideas have emerged that are discussed next in Chap. 4.
Synaptic contacts take place in dendrite spines which are small sac-like organelles projecting from the dendritic trunk. Dendritic spines are more abundant in highly arborized cells, such as pyramidal neurons. The number of excitatory inputs can be linearly correlated with the number of spines present in the dendrite. Dendritic spines contain ribosomes and cytoskeletal structures, including actin and tubulin proteins. The dynamics of cytoskeletal structures is central to our contention that information can be processed and delivered to the synaptic function by changes in the cytoskeletal structures of neurons [3].
1.3 How Experience Strengthens Synapses
To figure out how neuronal connections change, we have to know something about the mechanisms of action. A neuron communicates with another neuron by sending a chemical signal across the synapse. Depolarization of the terminal axon by the action potential causes the release of a chemical substance (neurotransmitter) in small multimolecular “packets” from the nerve terminal into the intercellular space. The neurotransmitter diffuses to the next neuron, usually separated by a gap (synaptic cleft) about 20–30 nm deep, and there it binds with the receptor projected from the membrane of the postsynaptic neuron. This binding leads to permeability changes in the membrane of the postsynaptic neuron. Depending on the chemical structure of the neurotransmitter and the chemical nature of the receptor substances (sites), the induced permeability change of the membrane leads to either excitation, i.e., it leads to initiation of an action potential, or inhibition, preventing or depressing the ability of a neuron to discharge. On most neurons there are both inhibitory and excitatory synapses, the balance of their influence determining whether a neuron will or will not generate an action potential or whether its frequency of discharge will increase or decrease.
Interestingly, the basic mechanisms for excitation and inhibition are similar. In both types of chemical synapses, transmitter substance is secreted by nerve terminals, and in each the transmitter increases the permeability of the postsynaptic membrane for selected ions only. At excitatory synapses the transmitter increases the permeability to certain cations (Na+ and K+) whose movement inside the cell will reduce the membrane potential and cause current flow which sets up impulse propagation. At inhibitory synapses the transmitter increases permeability for small ions (K+ and Cl−) which keeps the membrane potential below threshold and thereby prevents impulse propagation.
Whether a neuron will cause excitation or inhibition in the next neuron in line cannot always be attributed to the chemistry of the transmitter alone. Differences in the properties of the receptor may be equally important. For example, the motor nerve terminals at skeletal muscles secrete acetylcholine which combines with specific receptors at the motor end-plate. This leads to a simultaneous flow of Na+ and K+ ions across the membrane in the end-plate, causing a depolarization and conducted impulses, which in turn lead to an activation of the contractile mechanism in the muscle. In the heart the same neurotransmitter, acetylcholine, liberated by the nerve endings of the vagus nerve, causes an increase in the permeability for K+ alone, leading to inhibition.
Synaptic strengthening requires the participation of both neurons forming the synapse. Both the signal-sending presynaptic neuron and the signal-receiving postsynaptic neuron have to fire simultaneously in order to result in a stronger synapse. If the presynaptic neuron discharges and sends an electrical potential signal, but the receiving neuron does not respond, strengthening of the synapse does not occur. Or if the postsynaptic neuron discharges in the absence of presynaptic neuron firing, the synapses between the two neurons are not strengthened [2, 16]. Synapses do not strengthen willy-nilly because scattered neurons fire. Information must actually be transferred from sending to receiving neuron to ensure strengthening of the synapses. This requirement implies that the neural circuit must be functionally active to result in synaptic strengthening [17, 18].
Hebb [16] suggested that the more a synapse is used, the stronger and more efficient at transferring information it becomes. Use in turn is driven by experience. Thus, the same synapse may undergo graded weakening or strengthening according to its use, the type of stimulation and the availability of key chemical molecules, such as neurotransmitters [2].
The results of experiments by Bliss and Lomo [17] suggest that experiences strengthen synapses of only the specific pathways processing the experience; experiences do not affect synapses that are not activated by the action potentials. Thus specificity is built into the process. Oral experiences use specific circuits and synapses, thereby strengthening those very synapses and pathways, resulting in heightened input and processing of information in the cerebral cortex [3].
Hebb [16] speculated that learning and memory of experiences occurs at the synapses. He proposed that learning consisted of strengthening of synapses of a pathway. Strengthened synapses bind the neurons together into “ensembles.” If individual neurons represent different features of a scene, for example, the ensemble recreates an image of the scene in its entirety, like the pieces of a puzzle, resulting in learning.
1.4 Developmental Agents of the Brain
The other revolutionary discovery is that growth factors which normally reside in the body, are also present in the brain [19, 20, 30]. The growth factors are critical brain signals that strengthen the neuron connections, the synapses, enhancing then the transmission of electrical impulses in the brain [21, 22]. Brain growth factors convert experience into strengthened neuronal synapses that mediate learning and memory [2, 30]. Entire families of growth factor molecules, including nerve growth factor (NGF), epidermal growth factor (EGF), fibroblast growth factor (FGF) and brain-derived neurotrophic factor (BDNF), have been found to be produced in the brain, and different populations of brain neurons have been found to depend on a certain growth factor for growth and survival [23, 24, 30]. The implications are profound. The brain can no longer be considered a static immutable, hard-wired structure, but is a vast reservoir of growth factors that direct growth, survival, and flexibility of neurons [19, 20].
For example, the presence of NGF in the environment of cholinergic brain neurons (which release acetylcholine) causes division of neurons and activation of survival genes, which ensure neuron survival. NGF deprivation inhibits neuronal division and activates suicide genes, which cause neuron death [25]. Similarly, adding NGF to cultured “Alzheimer neurons” has been shown to extend the life of neurons that normally die in patients with Alzheimer’s disease [26, 27].
In addition, it has been suggested that BDNF in the brain governs everything from vision to thought to consciousness, sensation, and movement of muscles. BDNF factor has also been found to support survival of cholinergic neurons that degenerate in Alzheimer’s disease, the dopamine neurons that degenerate in Parkinson’s disease and the spinal motor neurons that die in Lou Gehring’s disease. BDNF is most abundant in a key memory center, the hippocampus [30]. Accordingly, it has been suggested that the brain neurons are programmed for both survival and suicide. External chemical signals determine whether the survival program or the suicide program of neuron is turned on [28, 29].
The most important finding, however, is that NGF appears to cause growth of nerve fibers, which subsequently innervate their targets. For example, during development before any nerves have sent fibers to target cells, each individual target releases NGF like a radio transmitter sending forth waves in all directions. In response, distant spherical nerve cells extend fibers that grow towards regions of ever-higher concentrations of NGF, until the target cell is reached. Once the nerve fiber has reached and has innervated the target, it is assured of obtaining the NGF hormone and surviving. Then the NGF, which is synthesized by the target, is taken up by the innervating nerve terminal and transported back through the nerve process to the nerve cell body. This process is called retrograde transport because it goes from the nerve terminal to the cell body in the opposite direction to the action potential. When the NGF arrives in the cell body via the retrograde transport biological actions are initiated, namely division of neurons, survival of neurons, and formation of fibers and operational circuits in the targets. Thus, the neurons whose fibers succeed in reaching the target live as a result of contacting a source of NGF. This mechanism is part of morphogenetic cell death, which occurs during development and generates the sensory and motor maps in the somatosensory cortex [2, 204].
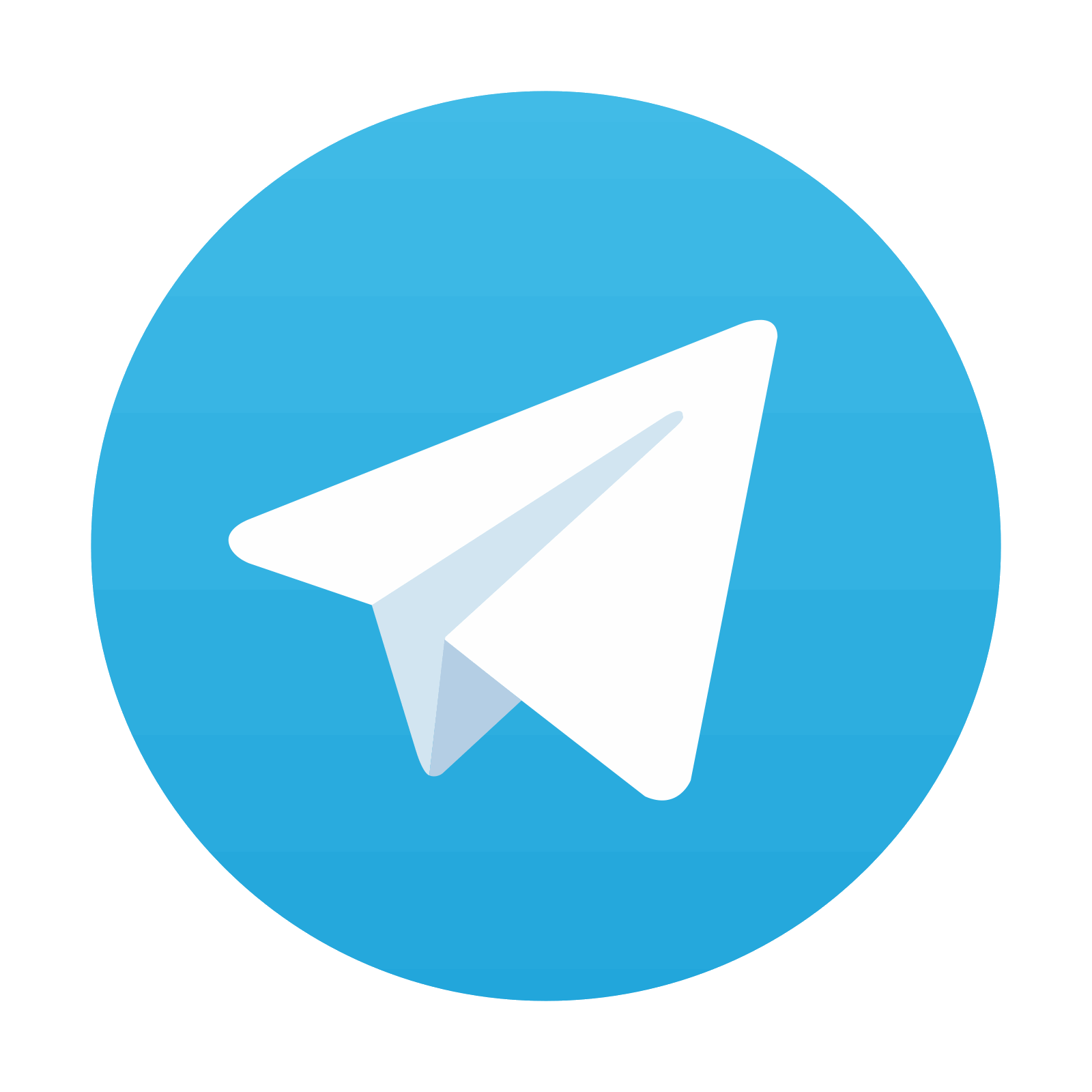
Stay updated, free dental videos. Join our Telegram channel

VIDEdental - Online dental courses
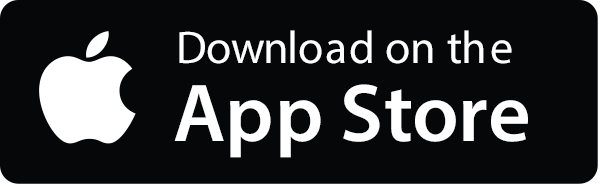
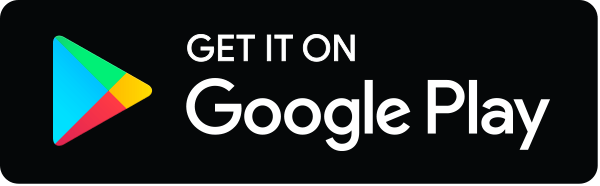