Fig. 1.1
The unit cell and simplified arrangement of hydroxyapatite, Ca10(PO4)6(OH)2
Biologic apatites are the inorganic phases of calcified tissues (teeth and bones). Similarity in composition of calcined bone to the apatite mineral was proposed by Proust and Klaproth in 1788 [4], and similarity in the X-ray diffraction patterns of bone and mineral apatites (HAp and fluorapatite (FA)) and similarity in composition (principally calcium and phosphate ions) led to the conclusion that the inorganic phases of bones and teeth are basically calcium hydroxyapatite [5–7]. Detection of carbonate associated with biologic apatites led to the speculation that these mineral phases are carbonate-containing apatites similar to the minerals dahllite (carbonate-containing apatite) or staffellite (carbonate- and fluoridecontaining apatite) [8]. Studies on synthetic carbonate-substituted apatites demonstrated that carbonate substitution in the apatite structure can proceed in two ways: CO3-for-OH or type A [9, 10] and CO3-for-PO4 or type B, coupled with Na-for-Ca [11, 12], and combined analyses of synthetic and biologic apatites using X-ray diffraction, infrared spectroscopy, and chemical analyses demonstrated that biologic apatites are carbonate apatites approximated by the formula (Ca,Mg,Na)10(PO4,CO3,HPO4)6(CO3,OH)2 [12–14]. For example, the following formula Ca8.856Mg0.088Na0.292K0.010(PO4)5.312(HPO4)0.280(CO3)0.407(OH)0.702Cl0.078(CO3)0.050 was proposed to describe the chemical composition of the inorganic part of dental enamel.
Early studies on synthetic apatites and related calcium phosphates were made to gain a better understanding of the structure, composition, and properties of biologic apatites, especially of human enamel apatites. However, studies on synthetic apatites in the last 30 years had focused on their preparation, their applications in medicine and dentistry, and their use as scaffolds for bone and teeth regeneration. Current commercial synthetic calcium phosphate biomaterials classified on the basis of composition include HAp, Ca10(PO4)6(OH)2; α- and β-tricalcium phosphates (α-TCP, β-TCP), Ca3(PO4)2; and biphasic calcium phosphate (BCP), an intimate mixture of HAp and β-TCP with varying HAp/β-TCP ratios [15–19]. Other commercial HAp biomaterials are derived from biologic materials (e.g., processed human bone, bovine bone derived, hydrothermally converted coral or derived from marine algae) [20–22].
This chapter is a brief review of biologic and synthetic apatites used as biomaterials, updating reviews published earlier by the authors [13, 20, 23, 24].
1.2 Biogenic Apatites
Normal and pathologic calcified tissues are composites of organic and inorganic phases. For bone, dentin, and cementum, the organic phase is principally collagen (about 25 % by weight) with smaller amounts of non-collageneous proteins [25]. On the other hand, the main organic phase in enamel is a non-collageneous protein (amelogenin) comprising about 1 % by weight of the enamel. In normal calcified tissues, such as in teeth and bones, in fish enameloids (teeth or calcified scales of some species), and in some species of shells, only carbonate (carbonate hydroxyapatite, CHA)- or carbonate- and fluoridecontaining apatite (CFA) occurs as the principal inorganic phase [13, 24, 26, 27]. In pathologic calcifications (dental calculus, urinary stones, vascular calcification, and other soft-tissue calcifications), biologic apatite may occur as one of the mineral phases that include other calcium phosphates, e.g., amorphous calcium phosphate (ACP), Cax(PO4)y; dicalcium phosphate dehydrate (DCPD), CaHPO4•2H2O; octacalcium phosphate (OCP), Ca8H2(PO4)6•5H2O; magnesium-substituted tricalcium phosphate (β-TCMP, (Ca,Mg)3(PO4)2); and calcium pyrophosphate dihydrate (CPPD), Ca2P2O7•2H2O [24, 28].
1.3 Enamel, Dentin, and Bone Apatite
Enamel, dentin, and bone apatite differ in crystallinity, reflecting crystal size (Fig. 1.2) and concentrations of minor constituents, mainly Mg and CO3 (Fig. 1.3a, Table 1.1) [13, 24, 29]. Enamel apatite contains the lowest concentrations of these ions and the highest crystallinity (larger crystals) compared to either dentin or bone apatite that shows much lower crystallinity (smaller crystals) or greater concentrations of Mg and CO3 (Table 1.1). These apatites also differ in solubility, decreasing in the order bone > dentin >> enamel.
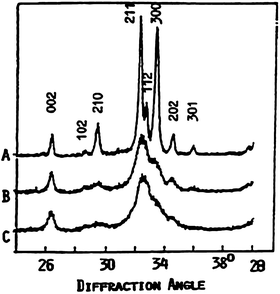
Fig. 1.2
X-ray diffraction profiles of biologic apatites from adult human enamel (a), dentin (b), and bone (c)
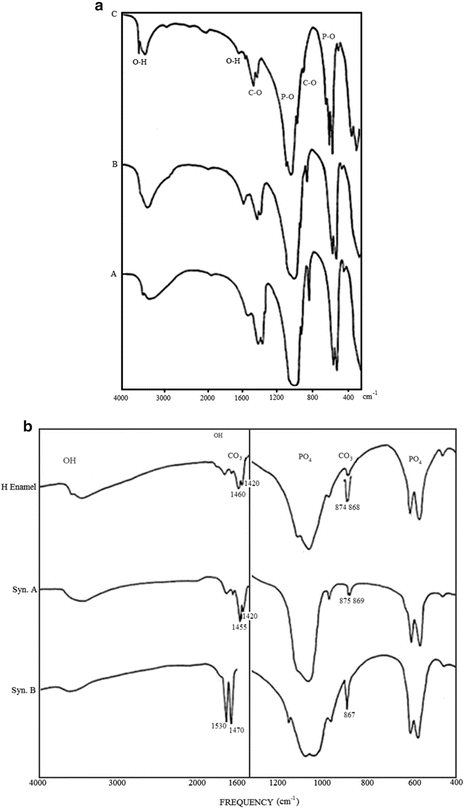
Fig. 1.3
(a) FTIR spectra of biologic apatites from adult human enamel, dentin, and bone. Note the higher resolution of the P–O (for PO4 groups) absorption spectra of enamel (C), compared to those of dentin (B) and bone (A) apatites. (b) FTIR spectra comparing the characteristic C–O (for CO3 groups) absorption bands in human enamel apatite compared to those in synthetic apatite with type A (CO3-for-OH) substitution (syn A) and with type B (CO3-for-PO4) substitution (syn B)
Table 1.1
Composition (%) and physical properties of apatites in adult human enamel, dentin, and bone
Enamel
|
Dentin
|
Bone
|
|
---|---|---|---|
Composition
|
|||
Calcium, Ca2+
|
36.5
|
35.1
|
34.8
|
Phosphorus, P
|
17.7
|
16.2
|
15.6
|
Ca/P (molar)
|
1.63
|
1.63
|
1.71
|
Sodium, Na+
|
0.5
|
0.6
|
0.9
|
Magnesium, Mg2+
|
0.34
|
1.23
|
0.72
|
Potassium, K+
|
0.06
|
0.05
|
0.03
|
Carbonate, CO3 2−
|
3.5
|
5.6
|
7.4
|
Fluoride, F−
|
0.01
|
0.06
|
0.03
|
Chloride, Cl
|
0.30
|
0.01
|
0.13
|
Pyrophosphate
|
0.02
|
0.10
|
0.07
|
Total inorganic (mineral)
|
97.0
|
70.0
|
65.0
|
Total organic
|
1.5
|
20.0
|
25.5
|
Absorbed H2O %
|
1.5
|
10.0
|
10.0
|
Trace elements: Zn, Cu, Fe, Sr, etc.
|
|||
Crystallographic properties
|
|||
Lattice parameters
|
|||
a-axis (+ 0.0003 nm)
|
0.9441
|
||
c-axis (+ 0.0003 nm)
|
0.6880
|
||
Crystallite size (nm, avg.)
|
33 × 3
|
2 × 0.4
|
2.5 × 0.3
|
Crystallinity index, b
|
70–75
|
33–37
|
33–37
|
Ignition products (800 ºC)
|
HAp + β-TCMP
|
HAp + β-TCMP
|
HAp
|
HAp, Ca10(PO4)6(OH)2
|
|||
a-axis = 0.9422 nm, c-axis = 0.6882 nm Crystallinity index = 100
|
|||
Composition: Ca, P, OH
|
These differences in crystallinity (crystal size) and solubility may be attributed to the differences in the concentrations of the minor constituents (e.g., Mg, N, CO3, HPO4). Studies on the effect of Mg and CO3 ions on the properties of synthetic apatites demonstrated that incorporation of these ions independently and synergistically causes the growth of smaller and more soluble apatite crystals [13, 24, 29–32]. The effects of CO3 incorporation on apatite crystal size and morphology and on dissolution properties are much more pronounced than that of Mg [13, 24, 32]. Proteins [25] and/or other ions (e.g., pyrophosphate, citrate) [12, 24] may also inhibit the crystal growth of biologic apatites.
Biologic apatites are usually calcium-deficient (i.e., with Ca/P molar ratio less than the stoichiometric value of 1.67 obtained for pure HAp, Ca10(PO4)6(OH)2). Calcining above 900 °C of human enamel and dentin apatite results in the loss of the CO3 constituent and formation of HAp and β-TCMP [13, 24]. Calcining of bone (human or bovine) above 900 °C results in the loss of CO3 and formation of mostly HAp with small amounts of calcium oxide, CaO [13, 24].
Partial dissolution of biologic apatites and precipitation of other calcium phosphates (DCPD, OCP, β-TCMP) are believed to occur in human enamel and dentin caries, characterized by the dissolution of the tooth mineral (carbonate apatite) by acids produced by oral bacteria [33, 34]. The non-apatitic calcium phosphates (e.g., DCPD, OCP) may, in turn, transform to apatites by hydrolysis or by dissolution and reprecipitation processes [13, 24, 34].
Biologic apatites have been idealized as calcium HAp, Ca10(PO4)6(OH)2[5–8]. However, the difference in lattice parameters: a-axis for enamel, 0.9442 nm vs. 0.9422 nm for HAp. Ca/P stoichiometry (e.g., about 1.63 for enamel or dentin apatite vs. 1.67 for HAp) and the association of other ions, notably magnesium (Mg) and carbonate (CO3) with biologic apatites (Table 1.1), have caused many years of disagreements and research on the structure and composition of biologic apatites [8, 9, 13, 35].
The nature of CO3 incorporation in biologic apatites, especially in human enamel apatite, had been a preoccupation of several researchers. The larger a-axis dimension of human enamel apatite compared to pure or mineral HAp was first attributed to the CO3-for-OH substitution (type A) in these apatites [9]. Such type of substitution was observed in synthetic carbonate apatites prepared by diffusing CO2 into HAp at 1,000 °C under very dry conditions, resulting in an expanded a-axis and contracted c-axis dimension B compared to pure HAp [9, 10]. However, studies on synthetic apatites prepared at much lower temperatures (60–95 °C) by precipitation or hydrolysis methods showed a partial CO3-for-PO4 substitution (type B) coupled with a partial Na-for-Ca substitution, resulting in a contracted a-axis and expanded c-axis dimension compared to CO3-free apatites [11–13]. The observed expanded a-axis of enamel apatite may be attributed to partial Cl-for-OH substitution [36] and HPO4-for-PO4 substitution [37] rather than the CO3-for-OH substitution in the apatite.
A possible H3O-for-OH was also offered as a possible cause for the expanded a-axis dimension [8]. Additional evidence for the dominant partial CO3-for-PO4 substitution in enamel and all biologic apatites is the similarity of the characteristic CO3 absorption bands between the IR spectra of enamel apatite with those of synthetic apatites exhibiting CO3-for- PO4 substitution as shown in Fig. 1.3b [13, 24, 38].
Studies in synthetic systems also showed that F incorporation (F-for-OH substitution) has the following effects: contraction in the a-axis dimension and no significant effect on the c-axis dimension compared to F-free apatites [13, 24], growth of larger and less soluble apatite crystals [13, 24, 39], and greater structural stability [3]. Such studies elucidated the nature of fluoride (F) incorporation in some biologic apatites (from modern and fossil teeth and bones). For example, the smaller a-axis dimension of shark enameloid compared to HAp or human enamel apatite is due to the high fluoride (F) concentration in shark enameloid (Table 1.1) [13, 27, 40]. The therapeutic use of fluoride in dentistry (sealants, topical gels, tablets) was based on the observation of low caries in areas of fluoridated water (1 ppm F) [41]. Fluoride treatment (topically or by the use of fluoridated dentifrices) of enamel and dentin leads to the formation of partially substituted (F, OH) apatite that is more resistant to acid dissolution, i.e., dental caries [29, 33, 34, 39]. Administration of F in the drinking water was shown to increase the crystal size and decrease the extent of dissolution of rat bones [42] and increase crystal thickness of enamel apatite [43]. Fluoride therapy (as NaF) has been recommended for the management of osteoporosis to increase bone density [44, 45].
1.4 Synthetic Apatites
Earlier extensive studies on synthetic apatites were made to gain a better understanding of biologic apatites and their properties. Because biologic apatite was idealized as HAp, most of the studies centered on HAp preparation and evaluation of HAp properties. Studies on synthetic apatites in the last 30 years were motivated by development of calcium phosphate-based biomaterials (principally HAp, TCP, or biphasic) for bone repair, substitution, and augmentation and as scaffolds for tissue engineering in bone and teeth regeneration. The rationale for developing HAp biomaterials was their similarity in composition to the bone mineral.
Synthetic HAp can be made by solid-state reactions or by precipitation or hydrolysis methods and subsequent sintering at high temperatures, usually 1,000 °C and above. Synthetic apatites can also be prepared using hydrothermal [46, 47], microwave [48, 49] or sol-gel [50, 51] methods. Apatite nanocrystals are obtained when prepared by precipitation or hydrolysis at lower temperatures (25–60 °C). Synthetic apatite crystals approximating the size of human enamel apatite may be obtained by precipitation or hydrolysis methods with reaction temperature, 80–95 °C (Fig. 1.4). Apatites may also be prepared in sol-gel systems [52], by electrodeposition [53, 54] or biomimetic precipitation on metallic or polymeric [55, 56] substrates.
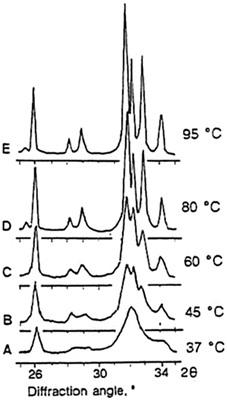
Fig. 1.4
X-ray diffraction profiles of precipitated apatites obtained at different reaction temperatures. The nanoapatite crystals similar to bone apatite are prepared at 37 °C (a) or at room temperature. The narrowing of diffraction peaks reflects increased crystallinity; the broader the diffraction peak, the smaller the crystals
Apatites obtained by precipitation involve the reaction of calcium salts (e.g., CaNO3, Ca(OH)2, CaCl2, Ca(Ac)2) and phosphate salts (Na−, NH4−, or K− phosphates) [24, 57]. Hydrolysis of non-apatitic calcium phosphates (e.g., ACP, DCPD, DCPA, OCP, β-TCP, α-TCP) or calcium compounds (e.g., CaCO3, CaMg(CO3)2, CaF2) in solutions containing OH, CO3, or F results in the formation of apatite, CO3− or F− containing apatites [24, 32, 36, 58]. Apatites prepared by precipitation or hydrolysis methods when prepared at pH between 5 and 9 are calcium-deficient apatites (CDA), and subsequent sintering results in the formation of BCP [59
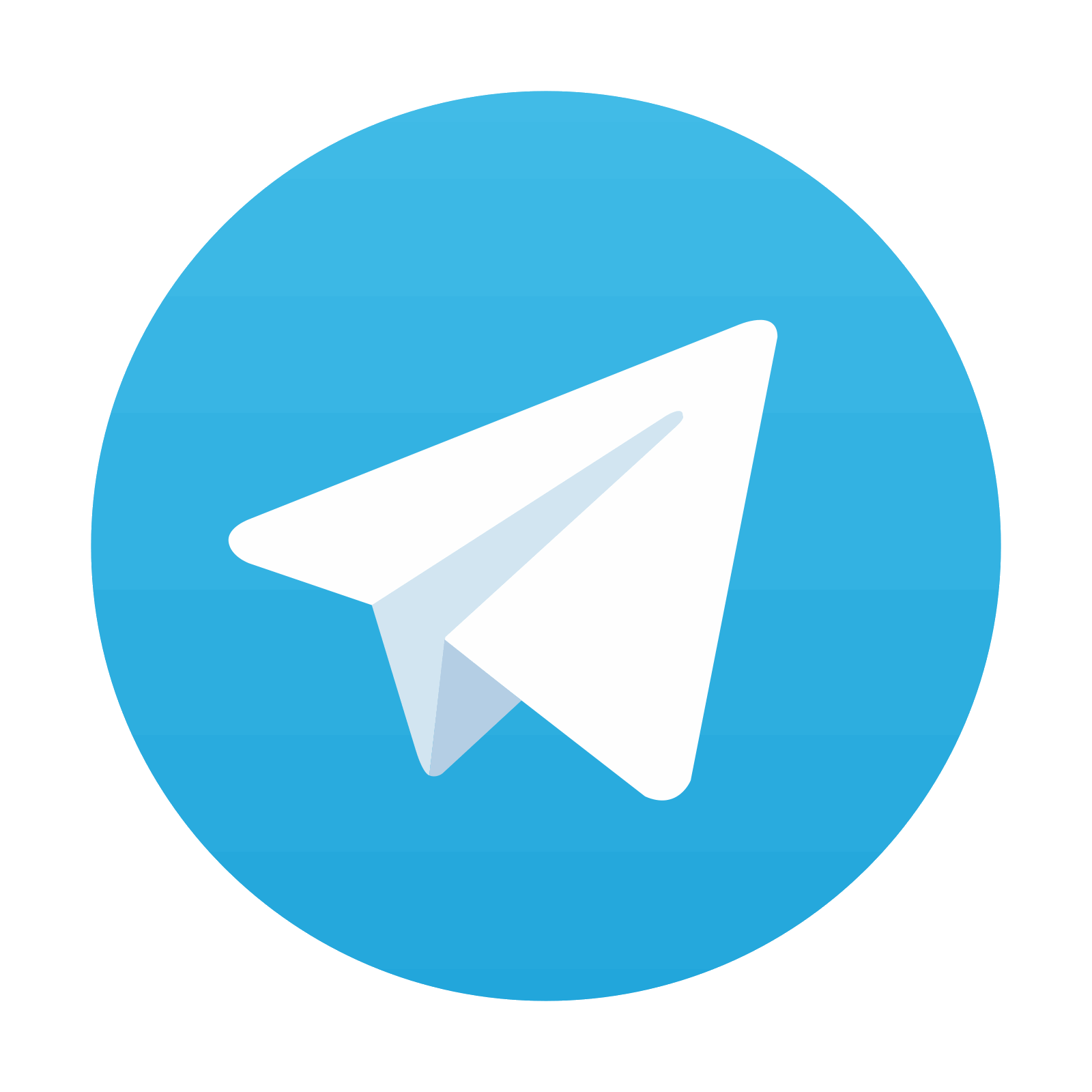
Stay updated, free dental videos. Join our Telegram channel

VIDEdental - Online dental courses
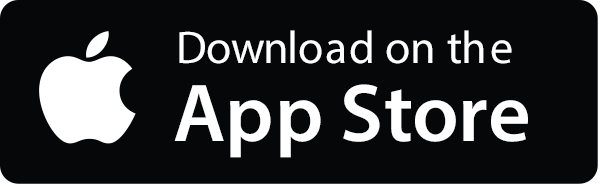
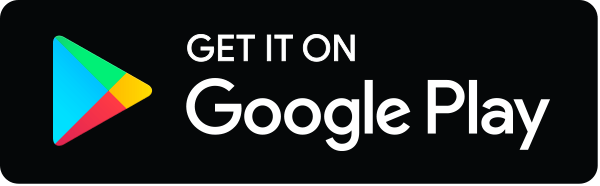