10
Gingiva and Periodontal Tissue Regeneration
Introduction
The fact that periodontal tissues possess the capacity, albeit minimally, to repair/regenerate, has led to substantial efforts focused on understanding the biological basis for this activity.
In the past few decades, various materials that can accelerate the reconstruction of periodontal tissues have been use. Matrix-based scaffolds, stem cells and growth factors have shown high potential for regenerative therapies. In dentistiy, such approaches have been explored to promote wound healing, restore periodontal ligament attachment, provide a broader zone of attached gingiva and cover the exposed root surfaces. Esposito et al. conducted a systematic review of soft tissue management for dental implants. They concluded that there is not sufficient evidence to provide recommendations for the optimal soft tissue augmentation technique. (1)
In the current concept, naturally occurring tissue regeneration can be reproduced in vitro. A tissue-engineering approach to utilize the regenerative capacity of stem cells residing within the periodontium (or other related tissues) involves the isolation of these cells and their subsequent proliferation/differentiation within a three-dimensional (3D) framework that could be surgically inserted in a given defect.
Anatomy
The tooth is attached to the jaw by a specialized supporting apparatus that consists of the alveolar bone, the PDL and the cementum, all of which are protected by the gingiva.
The mucosa immediately surrounding an erupted tooth is known as the gingiva. In functional terms, the gingiva consists of two parts: (1) the part facing the oral cavity, which is masticatory mucosa, and (2) the part facing the tooth, which is involved in attaching the gingiva to the tooth and forms part of the periodontium (Tencate 2017).
In an adult, healthy gingiva covers the alveolar bone and tooth root to a level just coronal to the cementoenamel junction. The gingiva is divided anatomically into marginal, attached and interdental areas. Although each type of gingiva exhibits considerable variation in differentiation, histology and thickness according to its functional demands, all types are specifically structured to function appropriately against mechanical and microbial damage (Carranza 2014).
The human gingiva is the oral muco sal tissue that surrounds the teeth and forms a mucoperiosteum covering the alveolar bone. Since the epithelial layer shows the capacity for continuous renewal, it is anticipated that this tissue could also be a source of stem cells.
Periodontal regeneration is a complex set of tissues and structures around the tooth; hence, an ideal biomaterial-driven approach should include a functionally-graded scaffold where the chemical composition and 3D architecture of each layer (or ‘compartment’) match the fine organization, biochemical structure, and mechanical properties of the tissues to regenerate (Giorgio 2019).
Periodontitis, an oral disease with a high prevalence worldwide, affects the function of teeth and constitutes one of the primary oral health burdens (Esposito et al. 2005). An epidemiological survey has suggested that more than half of all adults are affected by periodontal disease to varying degrees (Niklaus and Linde 2015; Iviglia et al. 2019) and a remarkable surge (25.4% increase) in the prevalence rates of periodontal disease was observed from 2005 to 2015 (Prichard 1957). Periodontitis can consistently disrupt tooth-investing tissues and lead to tooth loss if left untreated (Prichard 1957; Jepsen et al. 2002). Periodontitis is also closely associated with the occurrence and prognosis of various systemic diseases, including cardiovascular diseases, cancer, obesity, diabetes and chronic nephritis (Needleman et al. 2006; Trombelli and Farina 2008; Hammarström 1997; Giannobile 1996). Therefore, the exploration of effective and safe periodontal therapies that can be translated into the clinic is an urgent health need worldwide.
The ambitious purpose of periodontal therapy is to regenerate multiple periodontal tissues, including the alveolar bone, cementum and periodontal ligament (PDL) in the damaged periodontium (Whang et al. 1998). Although nonsurgical periodontal therapies (e.g., scaling and root planing) can prevent disease progression by physically removing the pathogens and necrotic tissues, only a small amount of periodontal tissue can be regenerated at the treated sites (Jepsen et al. 2002).
Overview of Past and Current Clinical Periodontal Regeneration Techniques
Past Techniques
The work of Pritchard (1957) provided early evidence that regeneration was not only theoretically possible but also clinically achievable in ‘ideal’ periodontal defects. This work showed that reconstruction of the periodontium in the apical aspects of three wall intrabony defects could be obtained following subgingival debridement through a surgical approach. Regeneration was not achieved in all cases, but this provided the ‘proof of principle’ evidence that if the conditions were optimal, then healing outcomes other than repair could be obtained (Prichard 1957). Since then, a great deal of research has focused on establishing a clinical technique to achieve these optimal conditions in a variety of clinical situations, not just three wall defects.
Guided Tissue Regeneration (GTR)
Guided tissue regeneration uses biocompatible barrier membranes to enable selective cellular recolonization of periodontal defects. The principle involves the use of a barrier membrane to exclude tissues that are unable to promote periodontal regeneration, such as the rapidly proliferative epithelium that grows along the root surface and the gingival connective tissues that fill the periodontal defect. Instead, this technique facilitates the repopulation of the defect with cells that can re-establish the periodontal attachment apparatus, namely those from the periodontal ligament and alveolar bone. The concept of GTR is supported by histological evidence of periodontal regeneration following the use of this technique (Jepsen et al. 2002). The membranes used can be either nonresorbable or resorbable, but due to decreased postoperative complications and a reduced number of surgical procedures, resorbable membranes have become more popular.
Resorbable membranes come in a variety of materials, with the most common being collagen and copolymers of polylactic/polyglycolic acid. The benefit of using GTR over the conventional use of open flap debridement has been reviewed in the literature, and the findings support the use of GTR in two clinical situations, Type II mandibular furcations (Jepsen et al. 2002; Needleman et al. 2006). However, although GTR is conceptually sound, the clinical results are unreliable and predictable regeneration is elusive in most periodontal defects, with the possible exception of a few ‘ideal’ situations, such as narrow three wall defects. These ideal clinical scenarios are not frequently encountered in clinical practice.
Platelet-Rich Plasma
The use of PRP, a platelet concentrate from autologous blood, is one of the strategies available for modulating and enhancing periodontal wound healing and regeneration (Iviglia et al. 2019). PRP includes a pool of GFs such as TGF-b, Vascular Endothelial Growth Factor (VEGF), PDGF, IGF-1, epidermal growth factor and FGFs. For this reason, it has been suggested that the use of PRP might increase the rate of bone deposition and bone quality in such dental treatments such as sinus lifts, placement of autogenous mandibular bone grafts, implants and periodontal surgery. Both preclinical and clinical studies demonstrate the effectiveness of PRP in bone and periodontal augmentation when used in conjunction with bone graft materials (reviewed in (Iviglia et al. 2019)). Unfortunately, the literature on the topic is contradictory and the published data are difficult to sort out and interpret. For example, in a series of clinical studies performed by Döri and co-workers on the healing of intrabony defects, the addition of PRP failed to improve the total outcomes in terms of probing depth reductions and CAL gains (Chang et al. 2009). Furthermore, PRP may not provide any additional effect when associated with GBR around dental implants (Bhatia and Ingber 2014). Although data published thus far suggest that PRP does not always exert additional effects, a systematic review of literature does give evidence for beneficial effects of PRP in the treatment of periodontal defects. Nonetheless, evidence for beneficial effects of PRP in sinus elevation appeared to be weak (Mao and Mooney 2015). While PRP has been somewhat beneficial when used in periodontal or implant regeneration, it has fallen out of favor recently because of the lack of controlled clinical trials providing strong evidence of its efficiency (Lee and Shin 2007). However, the use of a patient’s own biologically active proteins, GFs and biomaterial scaffolds for therapeutic purposes has opened a new way of understanding regenerative medicine. These simple and cost-effective procedures may have a potential impact in reducing the economic costs for standard medical treatments, soon achieving a ‘golden age’ by the development of user-friendly platelet concentrate procedures and the definition of new and efficient concepts and clinical protocols (Iviglia et al. 2019).
Growth Factors in Periodontal Tissue Engineering
Growth Factors and Biologically Active Regenerative Materials
The rationale for the application of growth factors to the periodontal defect is based on their ability to influence critical cellular functions such as proliferation, migration and differentiation. However, their use is not widespread in periodontal regenerative medicine, due to problems with dosage, rapid metabolic clearance, appropriate delivery and carrier systems and cost (Trombelli and Farina 2008). The most widely studied biologically active material that has been in widespread clinical use is enamel matrix derivative. Enamel Matrix Derivative (EMD), Enamel Matrix Proteins (EMPs) are secreted by ameloblasts and play a role in the regulation and growth of hydroxyapatite crystals that comprise enamel (Hammarström 1997).
The incorporation of bioactive molecules into periodontal biomaterials and scaffolds and their local and controlled release over time has been proposed as a valuable approach to obtaining osteoinductive implants (Giannobile 1996). Two different procedures to embed growth factors are available, i.e., either during the preparation of the material or after the fabrication (Whang et al. 1998; Fournier and Doillon 1996). Platelet-rich growth factor (PDGF), Bone Morphogenetic Proteins (BMPs) and Enamel Matrix Derivatives (EMDs) are among the most potent biomolecules for accelerating the periodontal wound repair in preclinical and clinical studies (Giannobile 1996; Barron and Pandit 2003; Taba et al. 2005; Lyngstadaas et al. 2009). Growth factors have the potential of stimulating the interaction between Mesenchymal Stem Cells (MSCs) and epithelial stem cells during tooth formation, alongwith all the following processes such as collagen formation, mineralized matrix deposition and fibroblast proliferation (Giannobile 1996; Giannobile and Somerman 2003). The morphology of the scaffold where signaling molecules are incorporated is the basis to achieve an extended and effective release. These are released by a diffusion mechanism which strongly depends on pore interconnectivity—of the material. The degradation properties of the graft may also affect the release rate of growth factors, which might be faster because of the high solubility of the matrix. Furthermore, how the carrier degrades—either by surface or by bulk degradation, resulting in a controlled or burst release, respectively—plays a key role, too (Tabata 2003; Yamamoto et al. 2003; Woo et al. 2001). It is clear that growth factors have a significant impact on tissue engineering outputs, which deserves to be explored.
Platelet-Rich Growth Factor (PDGF)
PDGF is the primary growth factor involved in wound healing, and there are a lot of studies showing its ability to enhance the proliferation and migration of periodontal ligament cells. It is naturally made of the conjugation of polypeptides of growth factor-BB and growth factor-AA, which is encoded by two different genes. It has been demonstrated that all isoforms have an effect on cell proliferation in vitro (Dennison et al. 1994; Oates et al. 1993). It has been shown that PDGF has a chemotactic effect, by which it can promote collagen synthesis, and stimulates hyaluronate synthesis by gingival fibroblasts and fibroblast proliferation.
Furthermore, if added to a culture with osteoblast-like cells, PDGF can regulate the ALP and osteocalcin expression (Giannobile et al. 1997). Lynch et al. (Lynch et al. 1991) applied PDGF in combination with the Insulin-like Growth Factor-1 (IGF-1) in dogs, and the results demonstrated significant effectiveness in periodontal regeneration.
Furthermore, the results of clinical trials revealed that the synergistic effect of these two growth factors could lead to the stimulation of bone regeneration inperiodontal defects in humans, too (Nevins et al. 2003). Even if used alone, PDGF can significantly stimulate the formation of new cementum and the production of collagen (Giannobile et al. 1996). Molecular cloning and large-scale purification have permitted the production of recombinant human PDGF, which has been mixed with TCP and has been made commercially available to clinicians (GEM 21s, Osteohealth, Shirley, NY, USA) (Izumi et al. 2011).
Platelet derived growth factor is a dimeric molecule produced by a variety of cells and tissues, including fibroblasts and osteoblasts (Antoniades et al. 1991; Hauschka et al. 1988). PDGF is mitogenic for various cells of mesenchymal origin, such as glial, smooth muscle and bone cells, as well as fibroblasts (Antoniades and Owen 1982; Ross et al. 1986). In fibroblastic systems, the primary effect of PDGF is that of a mitogen. It initiates cell division by acting as a competence factor, thus making the cell competent for division (Pledger et al. 1977). Platelet derived growth factor is a dimeric molecule produced by a variety of cells and tissues, including fibroblasts and osteoblasts (Antoniades et al. 1991; Hauschka et al. 1988). PDGF is mitogenic for various cells of mesenchymal origin, such as glial, smooth muscle and bone cells, as well as fibroblasts (Antoniades and Owen 1982; Ross et al. 1986; Stiles 1983). In fibroblastic systems, the primary effect of PDGF is that of a mitogen. It initiates cell division by acting as a competence factor, thus making the cell competent for division (Pledger et al. 1977), promoting mitogenesis of PDL cells (Bartold and Raben 1996). At concentrations of 10e20 ng/ml, in vitro studies suggested that PDGF-BB stimulated the proliferation of fibroblasts and osteoblasts (Hutmacher and Cool 2007; Woodruff and Hutmacher 2010), whereas a higher concentration (50 ng/ml) is required for the adhesion of PDL fibroblasts to diseased roots; concentrations of 5e20 ng/ml were not effective (Shue et al. 2012). PDGF has also been shown to be effective when combined with either IGF, as shown by significant bone fill on re-entry into the defects in animal models (Li et al. 2014) and in patients with periodontal disease (Zhang et al. 2009), or with dexamethasone (Inanc et al. 2009), the latter being a well-known osteogenic differentiation factor.
PDGF and IGF-1 had additive effects on calvarial DNA synthesis, but PDGF opposed the stimulatory effect of IGF-1 on collagen synthesis, and IGF-1 prevented the PDGF effect on collagen degradation (Hutmacher and Cool 2007). In further research, IGF-1 alone at a dose of 10 mg did not significantly alter periodontal wound healing, while PDGF-BB alone at the same dose significantly stimulated new attachment, with trends of effect on other parameters. For example, the PDGF-BB/ IGF-1 combination resulted in significant increases in new attachment and osseous defect fill at both 4 and 12 weeks (Bottino et al. 2011). In humans, the use of purified rhPDGF-BB mixed with bone allograft resulted in robust periodontal regeneration in both class II furcations (Vaquette et al. 2012) and interproximal intrabony defects (Carlo-Reis et al. 2011). Based on these studies, recombinant human PDGF-BB homodimer in b-TCP is approved for the treatment of intrabony and furcation defects, as well as gingival recession in periodontal disease and is commercially available as Gem-21 (Osteohealth Co., Shirley, NY, USA). Furthermore, a large multicenter randomized controlled trial study of PDGF-BB homodimer, together with b-TCP, in the surgical treatment of a 4 mm or greater intrabony periodontal defect demonstrated significant increases in Clinical Attachment Levels (CAL) reduced gingival recession at three months post-surgery, and improved bone fill when compared with those of b-TCP alone at six months (Carlo-Reis et al. 2011). The safety and effectiveness of this product was further demonstrated recently in a randomized, multicenter clinical trial involving 54 patients with periodontal osseous defects (Park et al. 2010). Although far from ideal for meeting the needs of complex periodontal therapy, the road from basic research to clinical applications of PDGF-BB, or BMP-2 suggests a potential use of protein-based therapeutics for stimulating and accelerating periodontal tissue healing and bone regeneration (Park et al. 2013).
Current Strategies
At present, the majority of tissue-engineering strategies applied to treat periodontal diseases claim at regenerating the alveolar bone through the implantation of suitable scaffold-based constructs. Specifically, periodontal regeneration relies on four basic paradigms (Dabra et al. 2012):
i) Implanted material (scaffold) acts as a three-dimensional (3D) template supporting new tissue growth;
ii)Cells are the primary building blocks of the reconstmctive strategies of periodontal tissue because of their proliferation and differentiation;
iii)Bioactive molecules (i.e., growth factors) promote cell activity that results in improved cell proliferation and differentiation;
iv)The blood supply delivers oxygen, nutrients and essential elements to tissue, and thus promotes the growth of newly formed tissue and helps to maintain homeostasis inside the 3D scaffold.
The healing process of the periodontal tissue is traditionally achieved by tissue repair; however, the advent of tissue engineering and regenerative medicine provides new possibilities in this regard (Shimauchi et al. 2013). Based on the definition, the repair process includes the healing of a wound by the growth of tissue that, however, may not fully restore the fine microstructure and, consequently, the function of the lost tissue (Lindhe et al. 1984; Wikesjö et al. 1992).
Two main objectives are simultaneously pursued in the current periodontal tissue engineering approaches, including (i) the stimulation of the growth of the key surrounding tissues by applying barrier membranes and bonegrafting materials and (ii) the prevention of the growth and proliferation of undesired cell types like epithelial cells (Melcher 1976).
The periodontium in its native form is a result of the interaction between mesenchymal and epithelial cells in the embryo (Palumbo 2011; Nanci and Bosshardt 2006). A series of independent and subsequent events are needed to complete the periodontal regeneration, which include osteogenesis, cementogenesis and connective tissue formation (Bei 2009). It has been well documented that various physico-chemical and mechanical stimuli can cause appropriate responses by the cells during the healing process. The type of cells used in regeneration controls the quality of the healing process, since they are initially responsible for repopulation of the wound (Melcher 1976). In the case of the periodontal regeneration, four distinct cell types compete—namely, periodontal ligament cells, alveolar bone cells, cementoblasts and epithelial cells.
Among these cells, the first three types are responsible for regenerating the periodontal tissue, while the last type—i.e., epithelial cells—are responsible for soft tissue regeneration. It is worth mentioning that the higher migration rate of epithelial cells (10 times faster) in comparison to the other periodontal cell types is the reason for observing the formation of the long junctional epithelium in the periodontal therapy (Engler et al. 1966). Infiltration of epithelial cells inside the defect can promote repair by the formation of an unusual architecture with a loss of function (Caton et al. 1987). Therefore, guided tissue membranes are implanted to limit the infiltration of the epithelial cells (Nyman et al. 1987). If epithelial cells are ruled out from the wound, other cell types with regenerative potential are thus allowed to become established, and epithelial down-growth can be successfully prevented (Linde et al. 1993). A combination of bonegraft materials, promoting the migration and differentiation of osteoblast cells, and GTR is the most commonly-used approach for achieving an optimal periodontal regeneration (Frost 1989a; Frost 1989b). Two reasons have been identified behind this synergic activity, i.e., the biological effects of bone grafts and the `Melcher hypothesis’, which explains the importance of cells used for the periodontal regeneration. According to this hypothesis, the origin of cells dictates the nature of the attachment in periodontal healing and the complete periodontal regeneration may be achieved when we apply cells with an origin from the periodontal ligament and the perivascular bone cells (Aurer and Jorgie-Srdjak 2005; Koop et al. 2012).
The biological actions induced by bone grafting materials can be divided into three interrelated healing processes: osteogenesis, osteoconduction and osteoinduction (Vuong and Otto 2002). The autologous bonegraft is the ‘gold standard’ option to achieve osteogenesis (Albrektsson et al. 1981). In this case, the undifferentiated and pluripotent cells derived from the transplanted material differentiate into the bone-forming cell lineage, i.e., osteoblasts, which will form a new bone (Burchardt 1983). Osteoconduction refers to the ability of a biomaterial to act as a suitable surface for guiding the bone growth by pre-existing preosteoblasts/osteoblasts (Vuong and Otto 2002; Hojo et al. 2009). Osteoinduction is usually identified as a specific property of materials regarding the induction of the differentiation of the host osteoprogenitor cells into osteoblasts (Fürst et al. 2007). In this case, the graft material excludes the undifferentiated connective cells and induces the differentiation and proliferation of the osteoprogenitor ones, thus leading to new bone formation (Vuong and Otto 2002; Quilynen et al. 2002; Price et al. 1996). The Melcher hypothesis also points out the importance of barrier materials for enabling cell migration from the connective tissue to avoid the repair process (Linde et al. 1993; Frost 1989b; Adeli and Parvizi 2012). Numerous previously performed animal studies clarified the efficacy of non-resorbable and resorbable membranes for GTR (Frost 1989b; Adeli and Parvizi 2012). Three correlated steps are involved in the healing process of periodontal tissue injuries. The first step is the epithelization of the inner face of the flap that forms the so-called long epithelium attachment. The maturation of the connective tissue is the second step in which we observe the formation of the so-called connective attachment. The final step is related to the recovery of bone architecture and the periodontal ligament at the level of the alveolar bone and at the deepest point of injury.
The morphology of the structure of newly formed tissue indicates whether repair or regeneration took place (Shimauchi et al. 2013). In the case of repair, fibroblasts mostly are involved in the healing process instead of osteoblasts. Thus the deposition of the osteoid matrix and new bone formation are inhibited (Lindhe et al. 1984; Wikesjö et al. 1992). On the other hand, regeneration involves the complete recovery of both the structure and the function of the periodontal tissue (Shimauchi et al. 2013; Koop et al. 2012). Despite the implementation of this approach, the success of periodontal regeneration depends on the capacity to control local infections, that are caused by microbial pathogens contaminating periodontal wounds (Vuong and Otto 2002; Pihlstrom et al. 2005; Zimmerli et al. 2004). The removal of necrotic tissue or a zone with acute infection are often identified as the major complications of periodontal defects and are high-risk points for bacterial growth and re-infection of new tissue (Hojo et al. 2009; Fürst et al. 2007). Hence, there is a need for implementing appropriate strategies to regenerate periodontal tissue and restrain bacterial growth (Quirynen et al. 2002). There are critical limitations for the currently-available strategies (e.g., conventional systemic antibiotic therapy) applied for reducing the risk of wound infection, including the systemic toxicity in the body which can lead to the need of hospitalization for monitoring (Price et al. 1996; Adeli and Parvizi 2012; Shahi and Parvizi 2015; Turgut et al. 2005). To overcome these problems, several research groups are working on advanced systems able to release a low dosage of antibiotics directly in situ (Ketonis et al. 2010; Zhao et al. 2009; Zilberman and Elsner 2008; Jose et al. 2005; Gristina 1987; Springer et al. 2004; Stigter et al. 2004). Promising approaches for avoiding bacterial adhesion and minimizing the side effects of systemic antibiotics include the coating of titanium implants with antiseptic molecules, the implant modification with functional groups exerting a bactericidal action, and the use of specific signaling molecules which selectively act as bactericidal substances.
Gene Therapy Approach in Periodontology
The critical drawbacks associated with the local delivery of growth factors include their short biological half-life in vivo as well as the high cost (Taba et al. 2005; Fang et al. 1996). Even more importantly, using a high dosage of bioactive molecules is needed to promote tissue regeneration, which could lead to unpredictable reactions and side effects; hence, an alternative approach to the local release of growth factors is the use of gene therapy for periodontal regeneration (Fang et al. 1996). Gene therapy involves the insertion of the genes of interest into an individual’s cells to obtain the desired functions, i.e., in most cases, upregulation of the expression of a specific growth factor (Sheikh et al. 2015; Barron and Pandit 2003; Nussenbaum and Krebsbach 2006). For this purpose, two main strategies have been proposed and developed including (i) in vivo technique, in which the gene vector is directly inserted into the target site (Fang et al. 1996; Schek et al. 2004), and (ii) ex vivo technique, in which selected cells are harvested, expanded, genetically transduced and eventually re-implanted (Gansbacher 2003).
Gene therapy has been applied for the upregulation of the expression of PDGF (Anusaksathien et al. 2003; Anusaksathien et al. 2004; Jin et al. 2004) and BMPs (Jin et al. 2003). In the in vivo technique, the gene of interest is directly delivered in the body, thus altering the normal expression of the target cells. On the contrary, the ex vivo technique involves the use of an adenovirus vector to introduce the genetic material into the target cells that have been harvested by a biopsy; eventually, transfected cells are re-implanted in the periodontal defect (Taba et al. 2005; Neel et al. 2014). In spite of the great potential of these techniques, there are several concerns about the safety of the adenovirus vector that still currently limit the clinical applicability of the gene therapy approach (Nussenbaum and Krebsbach 2006; Einhorn 2003).
Nanobiomaterials and Functionally-Graded Implants: The Last Frontiers in Periodontal Tissue Engineering
This valuable strategy is in its beginning in the entire field of tissue engineering—not restricted to only dentistry—and shows great promise for the 21st-century regenerative medicine. The existing examples of multi-layer scaffolds for multi-tissue regeneration often involve the use of nanomaterials, which can more closely reproduce the fine characteristics of the structure to regenerate (Kargozar and Mozafari 2018).
Sowmya et al. (Sowmya et al. 2017) recently reported the development of a 3-layer scaffold for the simultaneous regeneration of cementum, alveolar bone and periodontal ligament. Specifically, this scaffold was structured with chitosan/poly(lactic-co-glycolic acid) (PLGA)/nano-sized bioactive glass layer loaded with cementum protein 1 (CEMP1) for the cementum regeneration, chitosan/ PL GA layer loaded with fibroblast growth factor 2 for periodontal ligament regeneration, and chitosan/PLGA /nano-sized bioactive glass layer loaded with Platelet-Rich Plasma (PRP) growth factors for bone regeneration. Histological and tomographic evaluations showed that the implantation of this scaffold in rabbits led to complete periodontal healing and new alveolar bone deposition after three months
Incorporation of growth factors further stimulating tissue regeneration in nanomaterial-based constructs was also investigated. Zhang et al. (Zhang et al. 2015) fabricated MBG/silk fibroin scaffolds incorporating BMP-7 or PDGF-B adenovirus and implanted them in dogs. The scaffolds loaded with PDGF-B adenovirus were able to partially regenerate the periodontal ligament while those loaded with BMP-7 primarily improved new alveolar bone formation. The synergistic combination of these two growth factors promoted periodontal healing by allowing up to two times greater regeneration of the periodontal ligament, alveolar bone and cementum as compared to each adenovirus used alone.
Chen et al. (Chen et al. 2016) used a poly(caprolactone) (PCL)-based multiphasic scaffold, enriched with type I collagen, as a carrier to release CEMPl-loaded poly(ethylene glycol) (PEG)-stabilized amorphous calcium phosphate nanoparticles. After implantation in rats for 8 weeks, these scaffolds were shown to be able to stimulate cementum-like tissue formation but little new bone regeneration.
None of these scaffolds have been experimented in humans as yet, probably due to the lack of clear evidence about the effects of growth factors in the long term and the fate of nanomaterials used in vivo, as well as the high cost of the devices.
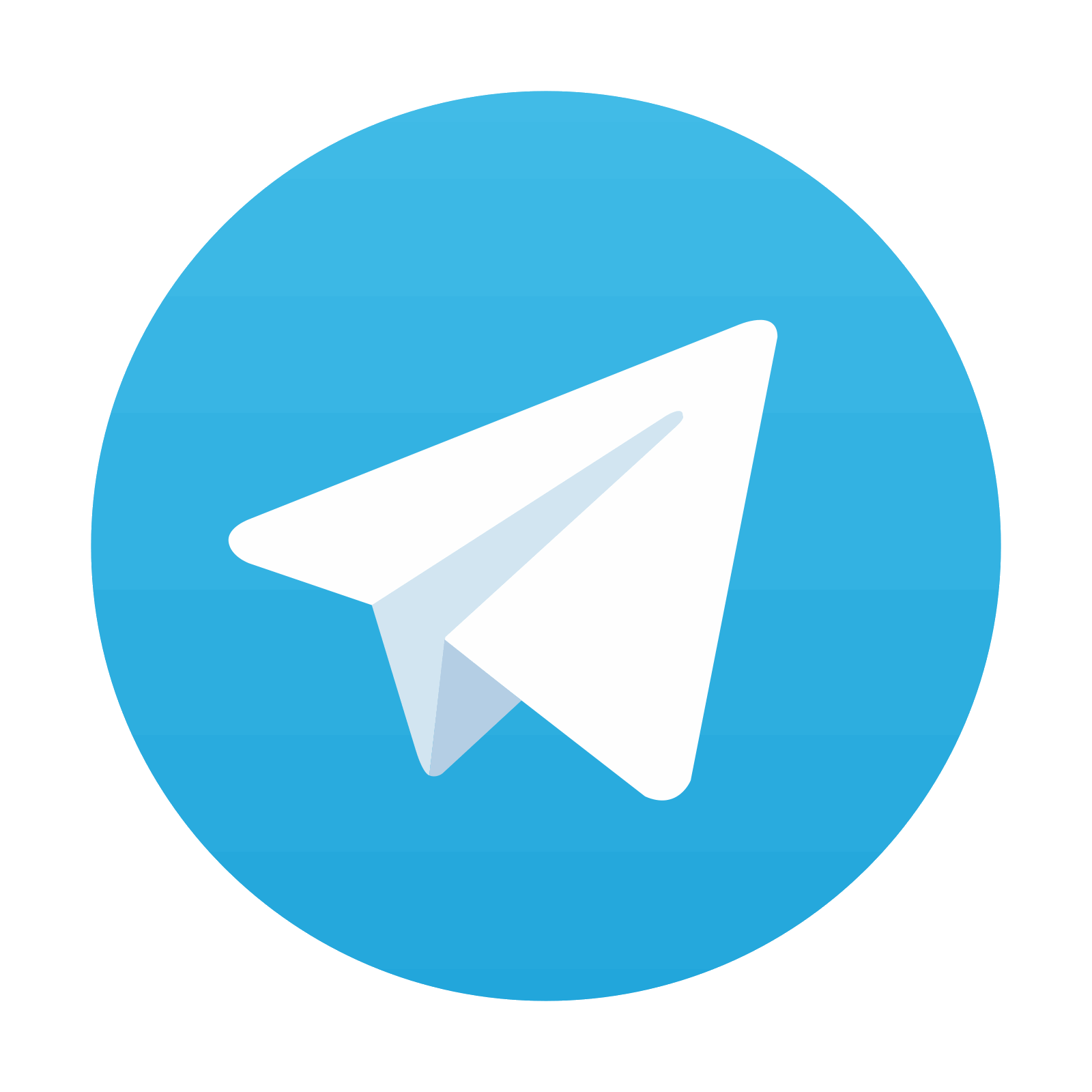
Stay updated, free dental videos. Join our Telegram channel

VIDEdental - Online dental courses
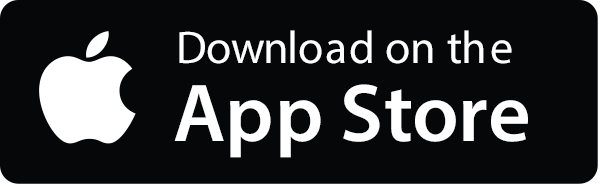
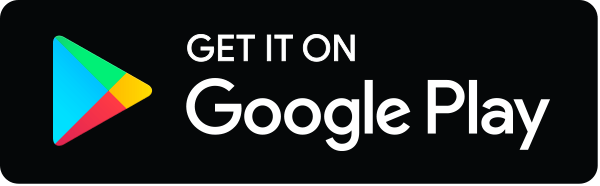