2
Translation of Tissue Engineering Approach from to Clinics
Introduction
Biotechnological advances in the dental and maxillofacial field have shown exponential growth in the last decade in terms of research funding and product development, resulting in novel more effective clinical applications. Now, more than ever, the industry is working with academia to produce new biomaterials allowing the possibility for techniques to either repair or regenerate biological tissues. The regeneration process is described as a slow replacement of a lost or damaged structure with the identical original tissue, a process observed naturally only during the first stages of life. On the other hand, repairing tissue is a much faster process involving the inflammatory cell cascade, following the deposition of a matrix and remodeling of the tissues in the damaged site.
Tissue engineering makes it possible for a clinician to repair or regenerate an affected tissue using cells fixed to biological or synthetic matrices or scaffolds which will guide the growth of the new tissue (Oakes 2004). In the near future, clinicians will be able to replace or regenerate completely lost or damaged oral structures with some kind of engineered product coming from a laboratory. Currently, scientific research is focusing on expanding our knowledge regarding the biological processes involved in tissue regeneration and repairment. This new knowledge has the potential to be translated clinically in more biological approaches for the final benefit of patients (Simon et al. 2011). Unfortunately, the commercially available products represent a small proportion of what has been tested and developed in the laboratory. The elevated costs and the complex regulatory pathway are responsible for the disparity between the amount of research investment and the number of products available in the market (Mishra et al. 2016).
New inventions and advances in tissue engineering commonly raise the same question among clinicians … WHEN? When will it be available in the market? And when can it be used in our clinical practice? It seems that other questions such as, how does it work? Who could benefit from it? And what adverse effects can we expect, play a secondary role. For researchers, it is impossible to predict when a new material or method will be available in the market, since the road from the laboratory to the final consumer is regularly a long way, full of complex challenges which not always depend on the research team’s efforts or capacity. Zafar et al., discuss some of the challenges in the process of translating tissue engineering research from the laboratory to the clinic. These challenges could be classified into two main groups: scientific and non-scientific challenges. Among the scientific challenges, they highlight the need for a multidisciplinary approach as one of the major concerns. Also, the complex nature of the oral tissues, the lack of an ideal scaffold material, and sterilization protocols are some of the challenges scientists and research groups must deal with in order to advance in bringing new therapeutic options to the clinic. On the other hand, regulatory and ethical issues regarding the use of some of the basic elements needed in tissue engineering like stem cells, funding opportunities, cost-effectiveness analysis, and the ideal packing and storage of these tissues are listed as the non-scientific challenges (Zafar et al. 2015). Most of the answers for overcoming these challenges are still to be discovered, others need a change in the perception of scientific progress from authorities and regulatory agencies. Now more than ever, the industry and academia need to work together, and scarce resources need to be used effectively.
Clinical Setting
Let us consider a likely real-life clinical situation. After cancer surgery, a patient loses a segment of the mandible and now needs the replacement of the lost bone structure. The treating clinician could opt for one of the available current options which include a standard titanium prosthesis or an autologous bone graft from a different donor site, for example Also, he may figure out a new therapeutic option, not yet properly tested or developed, which may bring more clinical benefits and fewer costs. After reading a press article, he thinks of a novel idea, a hypothetical 3D printable scaffold made of shrimp crust residues, which is also functionalized with an analgesic molecule to theoretically improve the postoperative application. As a clinician, the fundamental question still remains, how much time and effort would it take to turn this concept into an available commercial product ready to use?Figures 2.1 and 2.2 will lead us in exploring the pathway for how to turn this concept into an available product.
The Rise of New Ideas—The Key to the Laboratory
Tissue engineering has been defined as “an interdisciplinary field which applies the principles of engineering and life sciences toward the development of biological substitutes that restore, maintain or improve tissue function” (Langer and Vacanti 1993). This definition is valid to describe the concept of oral and craniofacial tissue engineering with a focus on dental and maxillofacial structures. As an interdisciplinary research field, this involves many scientific areas such as biology, mathematics, physics, chemistry, mechanics and informatics, among others. Hence, the final product is the result of the combined knowledge of these areas. In an attempt to simplify it, tissue engineering is a system mainly composed by a scaffold, cells and active molecules; consequently, every compound and also the combined compounds should be evaluated with in vitro and in vivo tests. The translation from the bench to the clinic consequently implies a hard-scientific work as well as an ethical challenge.
Translational research refers to all the steps needed for bringing the concept of an idea to advanced preclinical and clinical testing and, ultimately, to the development of new therapies for patients (Chen et al. 2012; Ungerleider and Christman 2014). As it can be exemplified (Fig. 2.1a), every new invention rises from a clinical need, and depending on the context, this clinical need can have different origins. In most cases, the main source of a clinical need is the patient itself who presents a certain disease or condition. Usually if there is an effective treatment available, the clinician will treat the patient with the existing approach; but, if this treatment is not ideal or could be enhanced, then the clinician should look for an alternative treatment. Some of the available ‘novel’ therapies may not fit every single case or may lack enough evidence to assure a safe and effective outcome. In such cases, the clinician may finally consider, once again, the traditional strategy. Of course, this will drag the limitations or side-effects already known, and the clinical need may still be unsatisfactory.

Figure 2.1. Schematic representation of the initial steps for the concept development process.

Figure 2.2. Translational flowgraph of the concept development process.
After recognizing the need for a new material, the clinician will play a key role in the translational process, which is to search in the market for available options; subsequently, they will turn to industry when they realize the material is not available (Fig. 2.1b). Let us consider again our example, the new 3D printable scaffold, which from now on we will call 3D-SCAFF! The treating clinician will search the literature and look in the market for a scaffold with analgesic purposes that also acts better than the available treatment options, just to find out that none exists yet. The market offers several kinds of bone grafts, but these are not ideal for this case. Then the market will ask the companies and industry if this product exists, with no positive response.
At this point, we can recognize a defining moment where the translational process could be interrupted. When the idea is presented to a pharmaceutical or medical business incubator or a startup company, the amount of resources the company invests in research and development (R&D) of new medical products and its experience in the regulatory process, is crucial (Bergek and Nounta 2008). For instance, a local or artisanal company might consider the idea interesting, but no further steps are taken since they do not have the capacity to develop the product. Nonetheless, if the company has an advanced program for R&D and usually invests money in testing new ideas, it could identify an emerging market and the possibility to create a new product that will strengthen its position in the market is conceivable. These kind of companies will make a deep analysis of the development opportunity, and if the project is considered profitable, then the translational process will continue, looking for either private or public sponsors to materialize the new idea. This is finally the key for the laboratory.
Activation of Scientific Resources—Where the Industry and the Academy Meet
The unavailable material has now spun from a problem to a proposal. The company in charge of the development process will shape the concept into a formal business-oriented document. At this point, hermeticism and confidentiality are mandatory, and conflict of interests should be analyzed. The patient’s welfare should always be prioritized and kept in mind as the driving force during this translational process. Other interests, such as financial benefits and professional prestige should be considered as secondary endpoints when taking a new product from the laboratory to the clinic (Bekelman et al. 2003). Now, the idea can be a mere intention of gaining the intellectual property of the invention, or in turn, it can worth millions of dollars if the product is finally developed. Secrecy and strict bioethical conducts between the different stakeholders are crucial when searching for the required scientific resources (Fig. 2.1c).
Biomaterial researchers need to provide meaningful endpoints to start the translation process (Tracey 2014), thus developing the idea should be scientifically and conceptually sound enoughto gain the trust of potential investors. The prospect to create a new product will depend on two key issues: financial and academic support. Even when a big company may cover both factors, usually the amount of human and economic resources needed can make the project unfeasible. Hence, the creation of interdisciplinary collaborations is decisive and should involve private and public contributors.
Public resources include the participation of the universities or academic public groups (such as government research facilities), which may be in charge of the experiments to develop the prototype of the desirable product (Fig. 2.1c). These institutions usually require the option of research grants to cover the expenses of the research, and most of these arrangements may require confidentiality agreements between the parties. In some cases, the academic groups themselves may play a leading role, creating an inner industry that will benefit the universities’ budget. This kind of participation is usually known as “spin-off companies”. Spin-off refers to how a company is created from another pre existing entity. In this case, entrepreneurial universities may play a key role in the economic and social development of a country (Miranda et al. 2018).
The private sector may contribute to the process as active developers financing the first experimental stages or only as mere suppliers of scientific resources. When time is vital and an unavailable advanced byproduct is needed, skilled suppliers are essential to minimize costs and time. By reaching out for these suppliers with specific technology and advanced manufacturing processes, the developers could access the required piece of the puzzle, avoiding side-developing investments (Martinelli-Lopes et al. 2015).
The 3D SCAFF is a good example to show this process. Once the industry identifies the need for this new scaffold, they may look at different scientific databases for a competent academic or research group, who will satisfy this demand This group could offer not only the equipment and technology but also the needed experience to guarantee an optimum beginning of the research process. If the developer recognizes that the project is too expensive to be covered by the company, the option of a public/private grant, to share the intellectual property with a sponsor or to activate a university spin-off company could be a good idea. Also, if the analgesic drug needed to load the 3D-SCAFF or a source of purified shrimp crust is needed, an outsourcer should be contacted to speed up the process. Once all the pieces are placed in order, the development process may begin (Fig. 2.1d).
Getting into the Laboratory
The concept development is ready to activate the laboratory protocols (Fig. 2.2a). This step, far from being a recipe to follow, is a complex multidisciplinary collaboration that will change depending on the desired product. For instance, some biomaterials for tissue engineering will require more mechanical than chemical evaluation, while others will need a deeper analysis of their biological behavior. However, one step cannot suppress the other, and a complete understanding of every factor is needed; from a full physicochemical characterization (Fig. 2.2a.1), to adynamic mechanical evaluation (Fig. 2.2a.2) and a biological guarantee of safety for further in vivo experiments (Fig. 2.2a.3). It is advisable for the research team to consult different international standards (such as ISO protocols) to assure that every step is sound and accepted by others.
Since all the experiments needed vary from one product to another, let us discuss the possible steps to develop 3D-SCAFF. First, all the single materials to be used must be characterized individually, determining their chemical composition, purity and behavior under different conditions (such as temperature changes, variable pH, pressure modifications or light exposure). Understanding each material will predict how they will behave once combined and may help to select and adapt the fabrication method. 3d printing—as an example—sometimes requires modifying the polymer temperature or the printing pattern; especially when an active molecule is added to the polymer. Once a possible combination is obtained, several mechanical tests will determine how the biomaterial will behave in the body and will predict the maximum load of force or deformation that may suffer. Different chemical analysis will also analyze the stability of the combinations, and the integrity of the added active molecules, such as drugs. Some vulnerable molecules may become inactive after preparation processes, so controlled release assays and bioactivity research must be conducted to determine if the loaded drug is still present.
Once some samples are obtained, the best laboratory options will be selected for biological tests to determine its biocompatibility (Fig. 2.2a.3). Biocompatibility states that the material must be non-toxic, non-allergenic, non-carcinogenic and non-mutagenic; and that it does not influence the fertility of the patient (Rogero et al. 2003). Specific experiments will determine the cellular response over the new product, in order to predict further responses from a living organism. In this context, the degradation and the by-products generated should be determined and understood as well. Although the first attempts to evaluate cytotoxicity depends on animal models, current advances in cell culture techniques have allowed the use of faster and standardized analyses to determine specific cell responses such as proliferation, adhesion, metabolism changes and differentiation, among others (Hanks et al. 1996). The obtained biological information; along with the mechanical and physicochemical data will lead to obtaining an accepted prototype: a theoretical ideal candidate (Fig. 2.2c).
Even when laboratory research is evolving, in vitro testing is essential to safely investigate the biological performance of newly developed devices when implanted in a living system. The animal model chosen should achieve the expected answers and should try to mimic the human response (Costa-Pinto et al. 2016). Unfortunately, a single animal model will only be used to evaluate a limited number of variables, and different tests and animal sources should be included to fully understand the biological response (Fig. 2.2d). In order to minimize the number of animals and tests required, the planning of each experiment must be meticulous, trying to obtain every possible data from each intervention. Life is valuable, and this does not exclude laboratory animals The design of the study should consider the aim of each phase (i.e., evaluation of bone response, determination of inflammatory reaction and/or related pain and expression of biochemical mediators, among others) as well as specific biomaterial features (size, shape, degradation time and byproducts) animal factors (species, age, sex, genetic background) and technical aspects (housing conditions surgical procedure, time of evaluation, sacrifice method) in order to guarantee a good experiment (Costa-Pinto et al. 2016; Pearce et al. 2007).
Contextualizing these steps to our 3D-SCAFF, specific experiments will include the characterization of both the natural polymer (including purity, stability, manipulation, physicochemical properties, etc.), and the analgesic drug. Compatibility between the materials and stability of the obtained product must be guaranteed before biological assays. Then, the cellular models will determine which of the prepared batches shows better behavior, and all data will then be translated into an animal model. In this case, an experiment to determine the bone reaction and replacement; and a second study to determine degradation and biocompatibility may be needed. However, as for in vitro biological assays, every experiment should have the corresponding bioethical authorization (Fig. 2.2b). It will be described that bioethical permission is not a license that allows a ‘free behavior’ in research. Single authorizations must be needed for every single step that involves living organisms; from samples to animals and of course human beings.
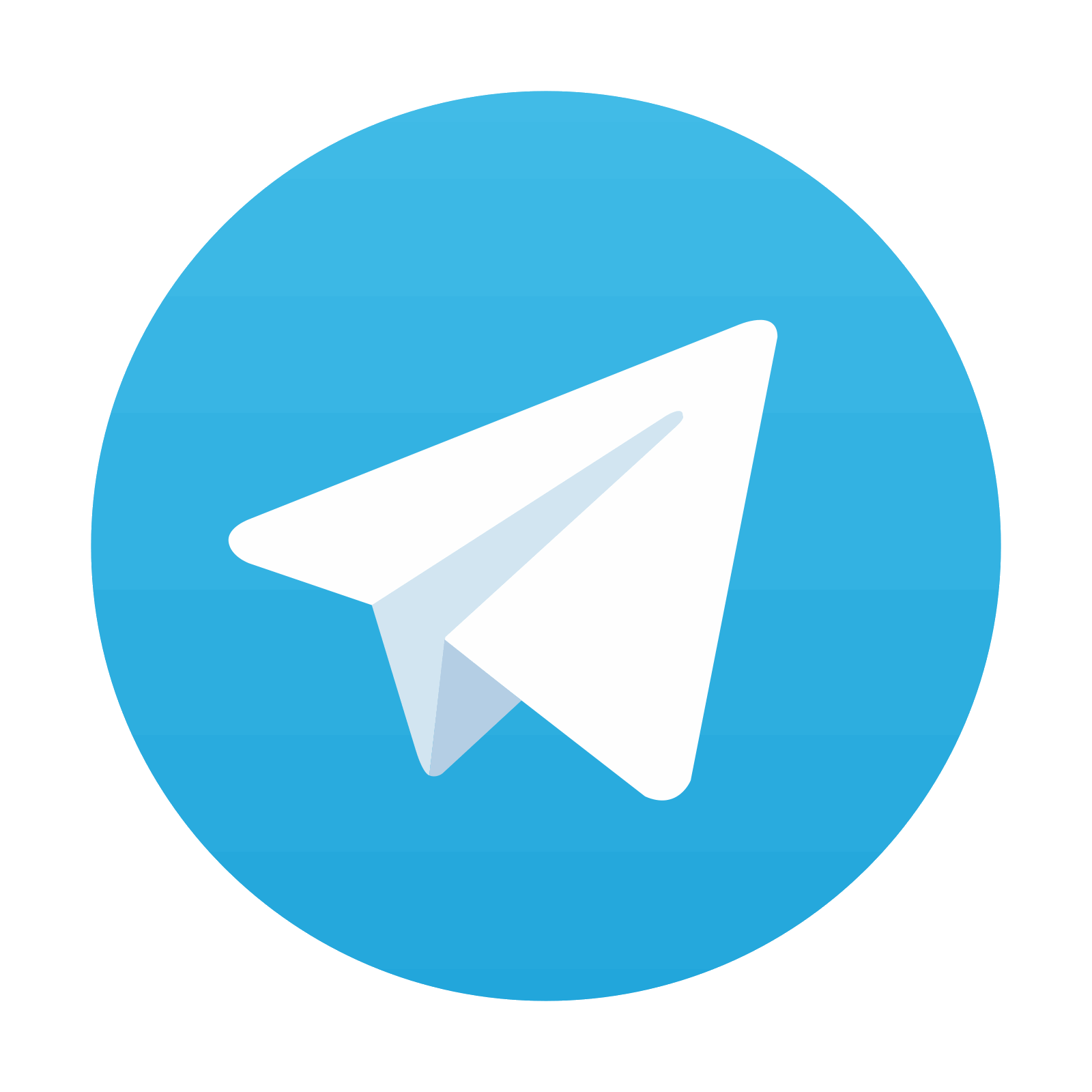
Stay updated, free dental videos. Join our Telegram channel

VIDEdental - Online dental courses
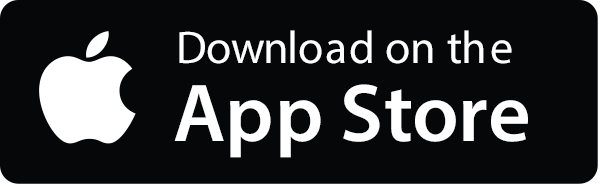
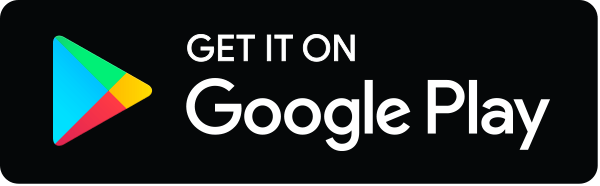