Calcium phosphate
Chemical formula
Occurrences
Apatite
(Ca,Z)10(PO4,Y)6(OH,X)2
Enamel, dentin cementum, bone, and dental calculi
Octacalcium phosphate, OCP
Ca8H2(PO4)6.5H2O
Dental calculi
Brushit, dicalcium phosphate dehydrate, DCPD
CaHPO4.2H2O
Dental calculi
Whitlockite, tricalcium phosphate, β-TCP
(Ca,Mg)9(PO4)6
Dentinal caries, salivary stones, and dental calculi
Our main focus in this chapter is on dental hard tissue-based calcium phosphates, which are mainly in the form of biological apatite. Dental hard tissue comprises enamel, dentin and cementum. The bulk of the dental hard tissue is dentin, which covers the dental soft tissue (dental pulp) lying at the core of the tooth. Enamel is the outer layer that covers the dentin in the crown area, and cementum is the outer layer, covering the dentin in the root area [2]. Enamel, as the masterpiece of biological mineralised tissues and bioceramics, has been of a great interest for material scientists and biologists. Specific features of enamel, including aesthetic, strength and durability, have inspired scientists to develop materials based on these nature-optimised designs. Natural beauty of enamel has inspired the development of direct and indirect tooth-coloured restorative materials. Resin composites and dental porcelain are the materials that have been developed to fulfil the growing demand for beauty and aesthetics in dentistry. Dentin has been a focus of research due to its well-designed hybrid structure, which represents an excellent biocomposite material. However, the most significant aspects of dental tissue structure, which are its exclusive mechanical properties and lifelong durability, have many features that remain to be clarified.
The need for functionally strong and durable dental materials capable of withstanding chronic mechanical, thermal and chemical stresses has created a long-term challenge for researchers in this discipline. The key to potentially unlocking this challenge is to gain an understanding of the magnificent structure and design of the naturally crafted (evolved) structure of dental tissues. The mechanical properties of dental tissues can be studied from two aspects: first, the effect of different compositional elements of this tissue on mechanical response of the tooth and, second, the role of structure and design of the building blocks of this tissue.
Among various methods that have been proposed for the study of mechanical features, nanoindentation has proven to be a reliable nondestructive mechanical testing method that enables the study of spatial variation of materials properties at submicron levels. A great benefit of this technique in studying dental hard tissues is its ability to investigate the materials with heterogeneous structures such as dentin and to some degree enamel. This ability of nanoindentation when coupled with recent structural analysis and microscopy techniques that reveal submicron structure of dental tissue has created unique opportunities to study the mechanical properties in relation to compositional and structural elements.
The aim of this chapter is to review the fundamental structure and properties of enamel, dentin and cementum, with an emphasis on their mechanical features. Most of the presented data are the results of nanoindentation studies on human dental tissue samples. Moreover, data from recent studies by the authors will provide new insights on the role of enamel matrix protein remnants and dentin matrix proteins on the excellent mechanical performance and durability of dental hard tissues. Furthermore, the effect of bleaching treatments, which has become a highly popular treatment of demand by patients, on the features and structure of the tooth will be discussed.
17.2 Structure of Dental Hard Tissues
Histologically, dental hard tissues include enamel, dentin and cementum. Enamel is a rigid, inert and acellular tissue that covers the tooth crown. Dentin forms the bulk of the tooth and as a tougher foundation provides enough support for the more rigid and brittle enamel [3]. In the root area, dentin is covered by cementum, which anchors periodontal ligament fibres that provide the support for a range of dental movements during mastication and function [2]. These tissues form a highly organised and complex structure with ideal functional and structural capabilities, which assist in sustaining mastication-induced mechanical loading and preventing their mechanical failures during function. For achieving a better understanding of these highly organised tissues, the structure and composition of enamel, dentin and cementum are reviewed in this section.
17.2.1 Structure of Enamel
Dental enamel, as the most highly mineralised tissue in the human body, consists of 96 wt% inorganic materials, which are mainly organised in the form of carbonated hydroxyapatite crystals. Other components of enamel include remnants of the organic matrix and loosely bound water molecules [2]. Enamel has an acellular and avascular structure without the ability to regenerate or repair itself. These features reflect the exclusive processes, which occur during the formation of enamel. Enamel formation (amelogenesis) happens during three primary stages, namely, cytodifferentiation, matrix secretion and maturation.
Amelogenesis is initiated by secretion of the organic matrix from the ameloblasts (enamel-forming cells) into the extracellular space adjacent to the dentinoenamel junction (DEJ). This protein-rich matrix controls enamel biomineralisation by self-assembling into supramolecular assemblies that initiate, regulate and organise the precipitation and growth of the hydroxyapatite crystals. Enamel matrix proteins are noncollagenous proteins composed of hydrophobic amelogenins and non-amelogenin proteins including ameloblastin, enamelin and tuftelin [4]. At the time of mineral deposition, enamel proteinases, enamelysin (MMP 20) and Kallikrein 4 actively participate in the selective degradation and removal process of the protein-rich matrix [5]. Throughout the long maturation stage, the initially protein-rich matrix of enamel is broken down by proteases and replaced by mineral deposition onto the pre-existing apatite crystals, allowing them to grow in width and thickness. After the maturation stage, enamel-forming cells (ameloblasts) disappear thereby preventing any further enamel deposition or repair [5]. This makes the preservation and care of dental enamel of paramount importance.
The result of the maturation stage of amelogenesis is a very hard, highly mineralised tissue, which consists of extremely long high-aspect-ratio hydroxyapatite crystals, and small amount of organic components and water. Enamel crystallites are principally composed of calcium and phosphorus as hydroxyapatite (HAp), Ca10(PO4)6(OH)2, with traces of sodium, magnesium, chlorine, carbonate, potassium and fluoride. The organic matrix of mature enamel constitutes 1–2 % of total enamel and functions as the glue for the apatite crystallites [2].
Structurally, the HAp crystals are tightly packed in three levels of hierarchical organisation, which explains some aspects of enamel’s mechanical strength [6, 7]. At the largest structural level, bundles combining dozens of enamel rods form the Hunter–Schreger bands. These bands are approximately 50 μm wide and are visible because of the different directions that adjacent bands of prisms reflect or transmit the light [8]. At the next level, enamel rods (prisms) and interrods (interprismatic substance) are visible which represent the primary and fundamental structural units of tooth enamel. Enamel rods are 5–8 μm in diameter cylindrical-like structures [9] that run continuously through the enamel from the dentinoenamel junction (DEJ) to the outer surface of enamel. Close to the DEJ, they are initially in a tortuous course, which follows the movement of the ameloblasts during initial cell growth and enamel deposition [2]. The diameter of the rods almost doubles from dentinoenamel junction to the surface of enamel [10]. In longitudinal section, each rod is near cylindrical in shape and made up of tightly packed apatite crystals with their long axis approximately parallel to the longitudinal axis of the rod (Fig. 17.1). A thin rod sheath surrounds each rod and separates it from adjacent rods by an interrod substance [11]. The line of demarcation between rod and interrod enamel can be identified by the change in crystallite orientation [2].
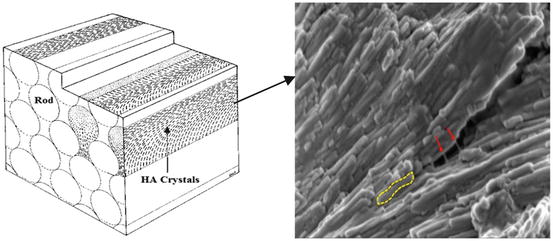
Fig. 17.1
Schematic of enamel rod and interrod structure (Modified and incorporated from Nanci [2]), composed of enamel proteins (red arrows) and HAp crystallites (yellow dotted line)
In cross section, enamel rods have a fish-scale- or keyhole-like pattern. The keyhole shape is made of a head and tail portions (Fig. 17.1). The head is rounded and defined by a thin proteinaceous rod sheath about 0.5 μm thick [2]. However, the rod tail is less defined and becomes continuous with the interrod enamel [9]. At the last structural level, the nanoscale level, each rod consists of extremely long carbonated HAp crystals which are thin ribbon-like structures with around 60–70 nm wide and ∼20 nm thick [12]. In the central part of the rod, the apatite crystallites are arranged with their long axis (c-axis) parallel to the longitudinal axis of the rod, whereas in the tail portion they diverge from the longitudinal axis of the rod by up to 65° [8]. Each calcium phosphate unit cell in the maturing enamel appears hexagonal. However, in fully mature enamel, the crystal outline looks more irregular due to the interaction with other growing crystals during the last stages of crystal growth.
Upon tooth eruption, although most of the enamel organic matrix is removed during mineralisation and maturation by the activity of enamel proteinases [5], some proteins are retained between the HAp crystallites and in the rod sheath [13]. In fact, some of the matrix proteins are resistant to protein degradation due to their strong binding to the HAp crystals and are retained in the mature enamel. These matrix proteins remain trapped between the crystallites and around the rods in mature enamel. This remnant matrix of mature enamel is a multicomponent protein/peptide mix formed by the association of different monomeric proteins [14]. Those proteins lying between crystallites have the function of ‘gluing’ HAp crystallites together, maintaining the hierarchical structure and enabling enamel to sustain the mechanical loading during function. The presence of remnant matrix proteins in mature enamel has been shown to influence its optical and mechanical properties. The minor components of enamel, protein remnants and water, have a profound plasticising effect [9]. Enamel is now known to be much more flexible and softer than its major component, crystalline HAp [15]. Structural changes in these protein components can reduce the ability of enamel to dissipate stresses [16]. In the later parts of this chapter, the effect of protein matrix modification of enamel on its mechanical response will be discussed. The presence of this organic matrix along with the mentioned hierarchical organisation of enamel has been shown to regulate the mechanical properties of enamel by forming a structure whereby flexural strength and elastic modulus decrease with increasing hierarchical dimension along with a change from linear–elastic to elastic–inelastic mechanical behaviour. This complex structural arrangement also makes enamel the hardest tissue in the human body [16]. Mechanical properties vary at different regions of enamel. The surface layer of enamel is stiffer, denser and less permeable than the rest of the enamel [8]. In spite of its hardness, enamel is extremely brittle especially when the underlying resilient dentin is lost.
Enamel is translucent and varies in shade from light yellow to grey white. At the incisal edge of a recently erupted tooth, enamel appears bluish white [11]. In cervical areas, enamel reflects the yellow colour of the underlying dentin, which determines the tooth colour along with the enamel thickness and translucency. The enamel translucency increases with age and therefore transmits the yellow colour of the underlying dentin and appears darker. Despite the fact that enamel is composed of tightly packed hydroxyapatite crystals, enamel is selectively permeable by way of the protein ‘glue’ structure to certain substances such as calcium and fluoride for remineralisation of demineralised enamel. Fluoride ions can penetrate the enamel from saliva and form fluorapatite crystal-rich layers at the surface, which are larger and more chemically resistant to bacterial acid dissolution [11]. Ions can penetrate enamel either from saliva, food and beverages, and then become incorporated into the interprismatic region or internally from the pulp through the DEJ and change its chemical composition [10]. In addition to small inorganic molecules, enamel is also permeable to larger molecules associated with stains and pigments. These large molecules diffuse through pores found at the prism boundaries and interprismatic enamel [8].
17.2.2 Structure of Dentin
Dentin and pulp tissue are generally regarded as a single functional and histological unit, termed the dentin–pulp complex [2]. However, we will only consider dentin in this chapter, as the subject of this book is about calcium phosphates. Dentin is a highly organised biological structure composed of complex protein assemblies and organised mineral components, which collectively form a rigid and durable mineral-rich biocomposite [17]. Dentin is formed through the process of dentinogenesis, by odontoblasts that differentiate from ectomesenchymal cells of the dental papilla. The dental papilla primarily induces the formation of dentin until it is finally surrounded by secreted dentin, thus forming the dental pulp [2]. A complex group of synchronised biological events regulates the formation and maturation of dentin. The main stages of dentinogenesis include the cytodifferentiation of the odontoblasts, the formation of mantle dentin, the control of mineralisation of the primary dentin organic matrix and, finally, the secretion of secondary and tertiary dentin. Primary dentin is the outermost layer of the dentin, which includes the mantle and the circumpulpal components. Secondary dentin is a layer that is secreted after the root formation. Tertiary dentin is the type of dentin that is secreted after full formation of the tooth, as a response to a stimulus, such as carious attack or wear.
Compositionally, dentin is a hydrated tissue comprised of approximately 50 vol% of carbonated hydroxyapatite minerals, 30 vol% of collagen and noncollagenous molecules and the remainder fluids. 90 wt% of the organic phase in dentin is almost exclusively composed of collagen type I, although other types of collagen have also been identified [18]. The remainder of the dentin organic matrix includes noncollagenous structures, of which proteoglycans (PG) are of a greater structural and mechanical importance [17]; other dentin matrix proteins, such as phosphoproteins and γ-carboxyglutamate-containing proteins, are believed to be more involved in mineral–matrix binding events [19]. Decorin and biglycan, two members of the small leucine-rich repeat (SLRP) family, are the PGs predominantly expressed in dentin. The most frequently found GAGs, in turn, are chondroitin 4-sulphate and a relatively lower content of chondroitin 6-sulphate.
Structurally, dentin is composed of packed dentinal tubules, measuring about 1–2 μm diameter surrounded by a hypermineralised layer called peritubular dentin, and a softer intertubular matrix, where the organic material is concentrated [20]. Dentinal tubules contain cytoplasmic extensions of odontoblasts, which lie in the pulp and originally were involved in the secretion of dentin matrix. In addition to this odontoblastic process, dentinal tubules contain dentinal fluid, which contains proteins and proteoglycans. The peritubular dentin is a highly mineralised composite material constituted of phosphorylated proteins [21, 22], proteoglycans and glycosaminoglycans [22], which lacks collagen fibrils [22, 23]. The intertubular dentin, on the other hand, is composed of supramolecular aggregates of collagen molecules, which are assembled into type I fibrils interconnected by noncollagenous components and water [24]. This array of organic molecules forms a hydrated organic network serving as a scaffold for the nucleation and growth of carbonated apatite mineral crystals [25]. The result of this organic–inorganic interplay is an intricate biocomposite that exhibits outstanding longevity and serves as a tough and resilient foundation for the brittle enamel, as well as a protective layer for the living pulpal soft tissue. This foundation prevents the propagation of catastrophic cracks from the brittle enamel further into the dentin [3, 26].
Dentin and enamel are bound strongly at their common interface, which is called the dentinoenamel junction (DEJ). Dentinoenamel junction is a hypermineralised area that appears like a well-defined scalloped area under the microscope. The presence and the form of this area have an important role in the structural and functional integrity of enamel and dentin. Due to the presence of dentinal tubules, dentin is far more permeable than enamel (Fig. 17.2).
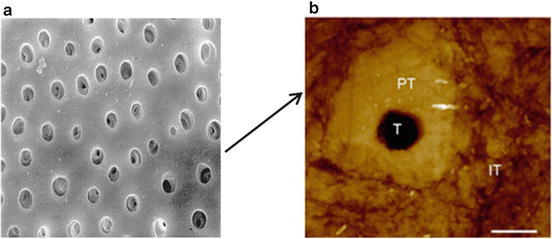
Fig. 17.2
(a) SEM of a transverse section of dentin. (b) Atomic force microscopy (AFM) image showing the cross-sectional view of the general structure of dentin tubule and peritubular and intertubular dentin (1 μm scale bar)
17.2.3 Structure of Cementum
Cementum is a mineralised avascular connective tissue, which is primarily composed of HAp minerals (65 wt%), with the addition of organic matrix (23 wt%) and water (12 wt%). The composition of cementum is very similar to bone. HAp minerals in cementum have a uniform small plate shape. The organic matrix is composed of collagen and noncollagenous proteins. Type I collagen fibrils comprise about 90 % of the organic cementum matrix. During cementogenesis, cementum is secreted by cementoblasts and after the completion of tooth development; it covers the roots of the tooth tightly in an interlocking manner. There are two types of cementum, which includes cellular and acellular cementum. Cellular cementum, which covers the apical third of the root, has an organised lacunar structure similar to bone and includes cementocytes. Acellular cementum covers the cervical portion of the root and has no organised structure and cells. It is assumed that cellular cementum anchors the periodontal ligament (PDL) fibre bundles and the acellular cementum has a more adaptive role [2]. Cementum, along with alveolar bone and periodontal ligament, forms the dental periodontium, which is the attachment apparatus of the tooth. Collagen fibrils within the cementum matrix play a crucial role in the attachment of periodontal ligament fibres to the tooth. The mastication forces exerted on the teeth are directed and distributed into the alveolar bone through these fibres.
17.3 Mechanical Properties of Enamel
Among various methods for studying the mechanical properties of dental tissues, nanoindentation has emerged as a nondestructive, precise method which is very useful in examining materials with small specimen size. Due to the differences which exist in the structure, mineral density, age and type among different teeth and also among different regions of each tooth, nanoindentation can provide a nondestructive method for studying a very small area of the specimen, therefore preserving other parts of the specimen for comparative studies.
Based on the load–displacement response of nanoindentation tests and its related calculations, several mechanical features of the test material can be measured. These parameters include hardness and elastic modulus, energy expenditure and creep behaviour.
In this chapter, we do not present the detailed calculation and theoretical basis for all of the nanoindentation experiments. Rather, we mostly emphasise on the presentation of the data and interpretation of the results which are of more interest for the readers of this chapter and are related to the scope of this book. Interested readers are encouraged to refer to available literature [27, 28] on nanoindentation and its application in studying biological tissues and other materials.
17.3.1 Mechanical Properties of Sound Enamel
In this section, we first discuss the basic mechanical properties of sound enamel, and later we will present the data on the contributing role of each structural element of enamel, including HAp, water and protein remnants in determining the mechanical behaviour of dental enamel.
17.3.1.1 Hardness and Elastic Modulus
Hardness is defined as the resistance of the material to permanent deformation under the indentation [29]. The nanoindentation hardness (H) is the contact pressure of the indenter divided by the projected contact area (A) of the sample at maximum load (P max) which can be estimated from [30]

(17.1)
Elastic modulus or Young’s modulus is the rigidity of a material within the elastic range. In nanoindentation experiments, the elastic modulus of the specimen E s can be calculated from [30]
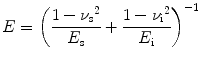
(17.2)
where E is the indentation elastic modulus (reduced modulus) and E i and v i are the elastic modulus and Poisson’s ratio of the indenter, respectively. v s is the Poisson’s ratio of the sample which is 0.3 for enamel [31].
Unlike the great variability in the results of hardness and elastic modulus tests of macroscale experiments, the results of nanoindentation tests show better consistency. However, these results depend on several factors related to sample properties and testing conditions. For example, the hardness for the top surface of enamel has been reported in a range of 3.39 ± 0.18 to 4.9 ± 0.5 GPa and the elastic modulus in the range of 83.4 ± 7.1 to 105.2 ± 1.3 GPa [27]. Lower values are reported for the cross-sectional surface [27]. Observed trends in the results of different measurements of hardness and elastic modulus can be summarised as follows:
1.
The hardness and elastic modulus of enamel are dependent on the direction of the load relative to its rod and interrod orientation [32]. The values are significantly higher at top (occlusal) surface of enamel compared with the cross-sectional lateral surface. These results are reflective of the anisotropic microstructure of the enamel. In fact, higher hardness and elastic modulus values are observed when the direction of the load is parallel with the direction of enamel rods and is resisted by thousands of aligned HAp crystals. Lower values are obtained when the force is applied perpendicular to the rods, allowing more displacement and movement due to the presence of less stiff organic sheathe.
2.
The hardness and elastic modulus are dependent on the site of the indentation within each tooth. Enamel is more mineralised in the surface and becomes less mineralised towards the DEJ [33]. The size of enamel rods is larger at the surface, while in the inner portion of enamel, softer interrods which contain more protein become larger and comprise a higher volume fraction of enamel. This difference in the amount of mineralisation and rod–interrod size is reflected in the mechanical features of enamel, as surface enamel has higher hardness and elastic modulus values compared with inner enamel.
3.
The elastic modulus is dependent on the type and size of the indenter tip and the applied load [34]. It has been suggested that with increased load of the indenter tip, more interrod area is involved in the indenter contact area which leads to inclusion of more organic sheath area with less elastic modulus value [27].
17.3.1.2 Stress–Strain Relationship of Enamel
The stress–strain curves of enamel and several dental materials were plotted from nanoindentation experiments [35]. There were several findings when comparing stress–strain curve of enamel with other materials. Firstly, the resultant curve for enamel is fairly smooth, and unlike pure HAp, no prominent elastic–plastic transition point is observed. This shows that enamel response to mechanical stress is different from that of HAp which is enamel’s main building block. This different mechanical response is attributed to the presence of organic component which makes the enamel a viscoelastic material.
Secondly, the surface perpendicular to the rod orientation shows a higher stress–strain response than lateral cross-sectional surfaces. This finding confirms the anisotropic nature of dental enamel and is explained by the response of protein-rich interrod area to mechanical stress.
Thirdly, the stress–strain curve of enamel is more similar to metallic materials than HAp and ceramics (Fig. 17.3). This behaviour of enamel is also caused by the presence of organic components and the hierarchical structure of enamel in different scale levels which imparts enamel with the ability to dissipate stress without losing its structural integrity by cracking.
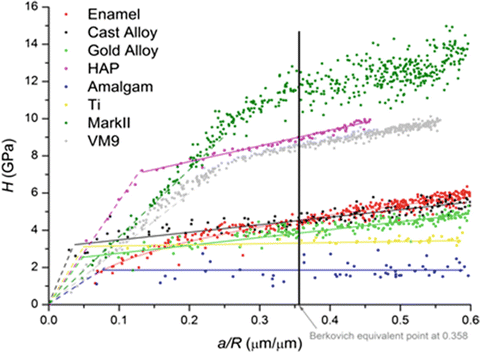
Fig. 17.3
Stress–strain relationship of different materials (solid lines show plastic part and short dots lines show elastic part of materials calculated from Hertz equation) [34]
17.3.1.3 Indentation Creep Behaviour
Inelastic strain of a material is caused by slow flow of its structure when it is held at a fixed load below its conventional yield strength for a period of time. This viscoelastic response may also be reversible, as is the case for enamel, which enables it to tolerate and redistribute the loading stresses during function [27].
The constant load indentation creep analysis is considered the most commonly used method for testing the creep behaviour of different materials including mineralised biological materials such as teeth [6]. With this method, the viscoelastic properties are recorded as the change in penetration depth with time under constant applied load. The difference between the displacement during creep at maximum load and recovery at minimum load represents the amount of unrecovered deformation during a hold period at constant force. In addition, the relative recovery of the material in relation to the deformation is calculated as a percentage by the relationship

(17.3)
where h 1 is the indentation depth displacement during hold at maximum load and h 2 is the displacement recovery during the holding time at a specific portion (typically 1 %) of the maximum load upon unloading.
This relative recovery indicates the amount of the deformation that occurs during loading and is recovered towards its normal state during a specific time interval upon unloading.
Comparison of the creep response of enamel, compared with HAp and dental materials, showed it exhibited a relatively stable creep response. In contrast, HAp did not show a viscous behaviour, and its creep deformation was very limited (Fig. 17.4).
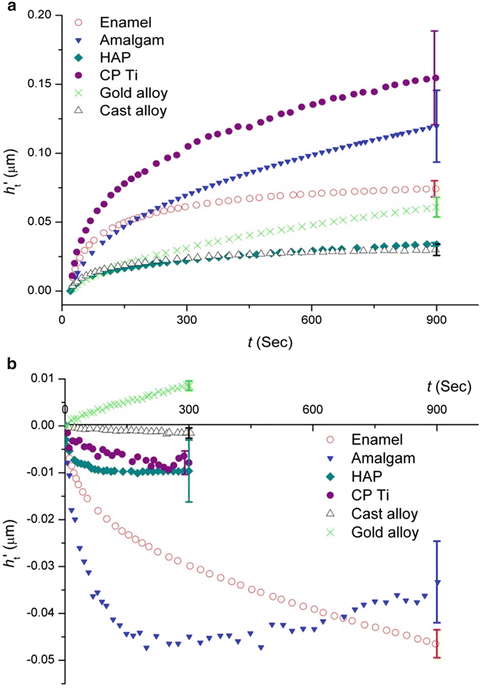
Fig. 17.4
Curves of (a) creep and (b) creep recovery of different materials. (h t′ values of amalgam in (a) were divided by 10 to fit onto the diagram.) (Reprinted from He and Swain [35]. Copyright 2007, with permission from Elsevier)
Similarly the response of enamel upon unloading was significant, while HAp exhibited limited creep recovery. The reversible creep behaviour of enamel is considered as a mechanism to withstand functional stresses during mastication which helps the teeth to distribute the localised high masticatory forces and sliding contact forces between teeth.
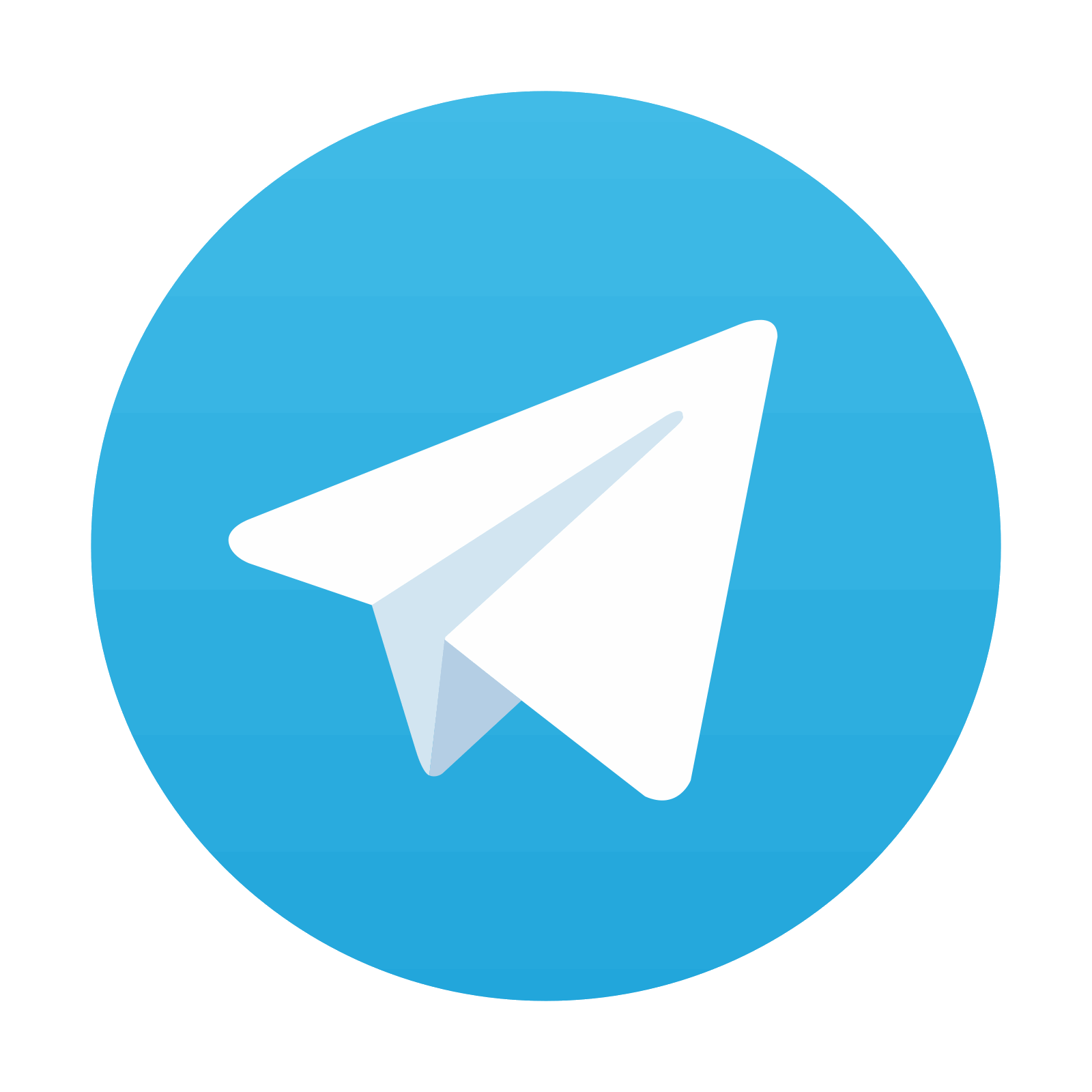
Stay updated, free dental videos. Join our Telegram channel

VIDEdental - Online dental courses
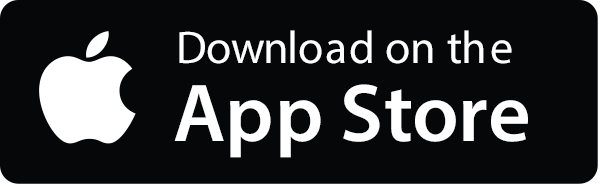
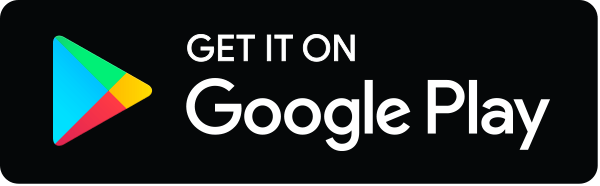