4
Brain Mechanisms of Oral Motor Functions
4.1 Introduction of Brain Mechanisms of Motor Control
4.1.1 Introduction
The importance of motor functions cannot be overemphasized. Through various actions, such as fight and flight, we can effectively adapt to the environment and increase the chance of survival. One of the major functions of the brain is to generate movements and acts (Wolpert et al. 2001). Notably, motor function is not a standalone function independent of other mental functions. It is closely associated with a variety of sensory and cognitive functions. For example, patients with Parkinson’s disease may show dysfunctions related to movement as well as cognitive processing (Kehagia et al. 2010). Therefore, to understand how complicated movements are generated and maintained, it is critical to investigate the brain mechanisms underlying the association between motor control and sensory and cognitive processing related to movements. In the following sections, we first introduce the concepts of motor control, motor learning and planning of movements. The role of cortical and subcortical regions related to motor functions is outlined.
4.1.2 Basic Concepts of Motor Functions
In daily language, two words related to human motor functions are usually used interchangeably: movement and act. From the point of neuroscience, a movement refers to a brief and discrete action that is confined to a specific body part. A good example of a movement is the simple reflex such as eye blinking or a movement for sensory orientation, such as turning one’s head towards an attractor (Rosenzweig et al. 2002). In contrast, an act refers to an assembly of simple and brief activities organized in a sequential pattern, such as swimming or driving a car. These acts consisted of movements of different body parts and are performed for a specific goal (Rosenzweig et al. 2002). The clarification of terminology plays a key role in clinical management. In terms of oral function, one should not equate eating, a complicated act, with jaw movement per se. As shown in the later chapters, the rhythmic movement of the jaw is essential to mastication, but mastication is associated with more complicated sensorimotor and cognitive–affective processing.
4.1.2.1 Motor Control
What are the primary goals for the motor system to regulate and control a movement or an act? That will be a good accuracy to achieve the desired goal and a reasonable speed to complete it (Rosenzweig et al. 2002). In order to achieve such a goal, the brain requires complicated processing of information related to motor control. A critical feature of motor control of the skeletal muscle system is its hierarchical architecture. Through a hierarchical ‘commanding’ system, motor commands are initiated from the cortex and relayed to the brainstem and the spinal cord, and further to the peripheral motor unit, i.e. a motoneuron and the muscle fibres the neuron innervates. The hierarchical architecture means that the element of a specific level serves its own role. For example, at the top level, the primary motor cortex (M1) gives a motor command to initiate chewing, but it does not directly control the muscle fibres in the masseter, which will be the task of the orofacial motoneuron. In contrast, the lower motoneuron directly controls muscle fibres rather than initiates the programme of movement.
The concept of motor control should be differentiated from motor planning, which broadly refers to ‘any process related to the preparation of a movement that occurs during the reaction time prior to movement onset’ (Wong et al. 2015). A critical element for a motor plan is to estimate the path of movement for achieving the goal (Wong et al. 2016). For example, upon swinging, baseball players need to estimate the trajectory of arm movement so that the ball can be hit. Therefore, the motor plan is established before the movement is executed. However, not all the mental functions related to movement execution are part of a motor plan. For example, to make a complex decision (e.g. ‘should I swing in this term?’) and to recognize task‐related objects (e.g. ‘what is the brand of the ball?’), which is not directly related to movements per se, are not considered part of motor planning (Wong et al. 2015).
4.1.2.2 Motor Leaning: Fine‐tuning of the Sensorimotor Association
From the perspective of behavioural adaptation, we are ‘learning’ things all the time. For example, when a new dental chair is installed, dentists will take some time to adjust themselves for the best posture to work with it. In other words, we need to accommodate the environment by digesting the sensory information and making an appropriate motor response. The motor learning system takes in sensory inputs and produces motor outputs (Wolpert et al. 2011), and motor learning refers to the process of refining the sensorimotor transformation so that individuals can adapt to environmental challenges (Wolpert et al. 2011). In order to emphasize the critical role of fine‐tuning between sensory and motor information during learning, the term ‘motor learning’ actually denotes the process of ‘sensorimotor learning.’ Several mechanisms of sensorimotor learning have been proposed in recent years, as summarized in the following sections.
4.1.2.3 Motor Programmes
While the concept of motor planning focuses on the preparatory process before movements start (Wong et al. 2015), the concept of a motor programme refers to ‘a set of muscle commands that are structured before a movement sequence begins, and that allows the entire sequence to be carried out uninfluenced by peripheral feedback’ (Keele 1968). This early definition highlights the role of programmed control on reproducing movements. Maintaining a motor programme is critical to the development of complicated movements that are usually acquired by learning. Critically, the relationship between a motor programme and motor learning is dynamic. A motor programme can be adjusted by receiving feedback according to the outcome of an action. Motor learning, which aims for adaptation of the environment, is associated with how motor programmes are modified.
4.1.3 Cortical and Subcortical Regions Associated with Motor Control
In the following sections, we provide a brief introduction of the cortical and subcortical regions associated with motor control. To present a bigger picture of the motor control system of human behaviour (not just for feeding), we start from the results from recent meta‐analyses of neuroimaging studies (Table 4.1) of a variety of movements and acts. (see Box 4.1 in the Companion Website) The key brain regions identified for the control system are discussed as follows.
Table 4.1 Meta‐analyses of neuroimaging findings of the motor area (since 2015).
Source | Studies | Methods |
---|---|---|
Worringer et al. (2019) | 18 fMRI/PET studies of dual tasking, 46 fMRI/PET studies of task switching |
ALE |
Gordon et al. (2018) | 42 fMRI studies of passive music listening | ALE |
Teghil et al. (2019) | 177 fMRI experiments of internally based and externally cued time processing | ALE |
Filgueiras et al. (2018) | 9 fMRI studies of kinesthetic/visual imagery in athletes or sportspersons | ALE |
Hardwick et al. (2018) | 303 fMRI/PET experiments of motor imagery, 595 experiments of action observation and 142 experiments of movement execution | ALE |
Lin (2018) | 20 fMRI studies of chewing or clenching | ALE |
Chang et al. (2018) | 67 studies (TMS/MRI/EEG/MEG/MRS/PET) of patients with chronic pain | Random effects model |
Winlove et al. (2018) | 40 fMRI/PET studies of visual imagery | ALE |
Ishibashi et al. (2016) | 56 fMRI/PET studies of tool‐related cognition | ALE |
Løkkegaard et al. (2016) | 18 fMRI/PET studies of sensorimotor tasks in patients with dystonia | ALE |
Tang et al. (2015) | 22 fMRI trials of interhemispheric activation balance in stroke patients with motor recovery | Random effects model |
Yuan and Brown (2015) | 14 fMRI/PET studies of drawing and 19 studies of writing | ALE |
Notes: ALE: activation likelihood estimation; EEG: electroencephalography; fMRI: functional magnetic resonance imaging; MEG: magnetoencephalography; MRI: magnetic resonance imaging; MRS: magnetic resonance spectroscopy; PET: positron emission tomography; TMS: transcranial magnetic stimulation.
4.1.3.1 Primary Motor Cortex (M1)
Traditionally, the M1 has been considered as the major component of cortical motor areas, which consists of the M1, the posterior parietal cortex, the primary somatosensory cortex (S1), the supplementary motor area (SMA) and the premotor cortex (PMC) (Figure 4.1). The M1 directly controls the motor system via the corticospinal pathway with the somatotopic organization that corresponds to our body. Therefore, the M1 is considered a critical element for movement execution. As predicted, the M1 activation is associated with the execution of a movement. This is evidenced by functional magnetic resonance imaging (fMRI) studies, which consistently showed the brain activation of the M1 during chewing and clenching (Lin 2018) and drawing and writing (Yuan and Brown 2015) (Table 4.1). Recent neuroimaging studies have disclosed a diverse role of the M1 in motor control (Table 4.1). Notably, the M1 activation is also associated with the condition when sensory processing plays a dominant role, such as the task of passive music listening (Gordon et al. 2018) and patients’ experience of chronic pain (Chang et al. 2018) (Table 4.1). When comparing motor execution and motor imagery (i.e. mentally rehearsing a movement without actually executing it), researchers found that the M1 played a dominant role during execution but not imagery. In contrast, the SMA and the PMC played a dominant role during motor imagery or observation (Filgueiras et al. 2018; Hardwick et al. 2018; Winlove et al. 2018). However, this distinction is not clear‐cut. For example, during motor execution, the brain activation of the SMA, the PMC and the M1 was noted (Yuan and Brown 2015; Lin 2018); during the preparatory phase, the brain activation of the M1, the SMA and the PMC can collectively predict the subsequent movement (Hirose et al. 2018). The findings suggest that the M1 forms a complicated network of sensorimotor processing with other components of the motor system.

Figure 4.1 An overview of brain regions associated with motor control. The figure only displays the relative position and size of the brain regions, not depicting the anatomical details.
The interaction between the M1 and other components of the motor system is further demonstrated by two recent studies using multivariate pattern analysis (MVPA). Park et al. (2015) reported that when subjects were performing a motor execution or imagery task (for hand grasping and hand rotation), brain activation at the M1, the PMC and the SMA were consistently found in all experimental tasks. The MVPA revealed that the activation of the M1 predicted the executed movement with the best accuracy, far beyond the SMA/the PMC. However, during the imagery task, the three regions showed a similar prediction accuracy to imagined movement in hand grasping, and the SMA showed the highest prediction accuracy to hand rotation. Furthermore, Hirose et al. (2018) reported the brain activation of a finger‐tapping task, in which the subjects were free to choose which finger would tap. They found that before tapping (i.e. during the preparatory stage), the brain activation of the M1, the SMA and the PMC (the PMd subregion, see below) collectively predicted the choice of which finger to tap (i.e. during the execution stage). The findings revealed that the M1 may participate in planning a movement. In sum, the recent findings suggest that the M1 plays a predominant role in motor execution but is not limited to execution per se. The M1 may also work closely with the SMA/the PMC during the preparatory stage.
4.1.3.2 Premotor Cortex
The human PMC resides in the dorsolateral area of the brain, just anterior to the M1 (Figure 4.1). The PMC is further differentiated in the more dorsal part, the PMd, and the more ventral part, the PMv. Both animal and human neuroimaging findings suggest that the rostral and caudal parts of the PMd are different structurally and functionally (Picard and Strick 2001). Anatomically, the caudal PMd has projections to the M1 and the spinal cord, with a close association with the motor area. In contrast, the rostral PMd does not show projection to the M1. Instead, it is functional and structurally more associated with the PFC (Picard and Strick 2001).
Functionally, the PMC activation has been identified in various stages of motor control, not confined to the period of movement execution. For example, the PMC activation was identified during dual tasking (i.e. performing two tasks concurrently) and task switching (i.e. performing two tasks concurrently alternatingly) (Worringer et al. 2019) and during visual imagery (Filgueiras et al. 2018; Winlove et al. 2018). Its activation was identified when one was imagining doing a movement or observing the other’s movement (Hardwick et al. 2018). The PMC activation is associated with the processing of using a tool (Ishibashi et al. 2016) and encoding short sequential elements for a movement (Diedrichsen and Kornysheva 2015). Notably, the PMC activation is also identified during a sensory task (Gordon et al. 2018), suggesting its role in integrating sensory and motor information. In an earlier study of 12 young adults, the researchers asked subjects to attend to moving objects with different focuses: to focus on the moving object per se, to focus on the spatial feature of the movement and to focus on the temporal feature of the movement (Schubotz and Von Cramon 2001). The results showed that the activation of the left superior ventrolateral PMC was stronger when focusing on the object, and the activation of the dorsolateral PMC was greater when focusing on the spatial features (Schubotz and Von Cramon 2001). The findings suggest that the PMC plays a key role in the processing of the sensory feature related to movement. Finally, meta‐analytic findings of patients with stroke have revealed that a good motor recovery is associated with the balance of interhemispheric activation of the PMC as well as the sensorimotor cortex, suggesting its role in motor learning (Tang et al. 2015) (Table 4.1). Re‐organization of the PMC is also observed in post‐stroke patients, which can be associated with the recovery of motor functions in the patients (Kantak et al. 2012).
4.1.3.3 Supplementary Motor Area
The human SMA resides in the dorsal medial wall of the brain, just anterior to the M1 (Figure 4.1). The SMA can be further differentiated into the pre‐SMA and the SMA proper. The pre‐SMA resides posterior to the PFC. While the SMA directly connects to the M1 and the spinal cord, the pre‐SMA connects with the PFC (Picard and Strick 2001). The pre‐SMA is only sparsely connected to the corticospinal system (Nachev et al. 2008). The SMA also holds the somatotopic organization, i.e. the movement of a specific body part will correspond to a specific region of the SMA (Nachev et al. 2008). The functional heterogeneity between the SMA proper and the pre‐SMA can be identified in neuroimaging research: activation of the pre‐SMA is more associated with the cognitive processing of motor action, which is also associated with the PFC.
Both the pre‐SMA and the SMA show some similar functions in motor control. For example, according to the results from imaging meta‐analyses, the activation of the SMA has been identified for executing an action, e.g. chewing and drawing/writing (Yuan and Brown 2015; Lin 2018), together with the M1 and the PMC. Moreover, just like the PMC, the SMA activation is consistently found during planning and control of a movement (Teghil et al. 2019; Filgueiras et al. 2018). While the PMC plays a key role in a movement cued by an external signal, the SMA is associated with self‐initiated movements (Rosenzweig et al. 2002). The SMA is associated with cognitive control of movement. Here ‘cognitive control’ refers to flexibly alter the ongoing action, such as stopping the current action or change from one action to another. For example, animal research has revealed that the pre‐SMA neurons responded to change of a different motor programme (Matsuzaka and Tanji 1996), and human neuroimaging research also reported that pre‐SMA activation was associated with changing or stopping a current motor task (Nachev et al. 2008). Abnormal sensorimotor activation of the SMA may reflect motor deficits. For example, patients with dystonia, i.e. an abnormal pattern of muscle contraction, showed decreased activation in the SMA and somatosensory cortex (Løkkegaard et al. 2016). All the findings highlight the role of the SMA and the PMC in the sensorimotor transformation for behavioural adaptation.
4.1.3.4 Basal Ganglia
The basal ganglia (BG) includes both a direct and an indirect pathway of control (Figure 4.2a). The direct pathway consists of the projection from the striatum to the internal segment of the globus pallidus (GPi) and the substantia nigra (SNr). The indirect pathway consists of the projection from the striatum to the external segment of the globus pallidus (GPe). The GPe then sends projections to the GPi and the SNr via the subthalamic nucleus (STN). The BG has an extensive network with the cerebral cortex and the cerebellum. It forms the corticostriatal circuitry: the projections from the striatum to the cortex (via thalamus) and the projection from the cortex to the striatum. Moreover, the STN neurons send projections to both the motor and non‐motor (Crus II) regions of the cerebellum (Bostan and Strick 2018). During motor control, both the BG and the cerebellum receive and send information to the M1 for executing a movement. Moreover, in terms of motor learning, the BG is widely considered a critical component of reward‐based learning, i.e. the learning process that optimizes performance via reward signals. The cerebellum, in contrast, plays a key role in error‐based supervised learning (see Section 4.4).

Figure 4.2 Sensorimotor control of the basal ganglia and the cerebellum. (a) The basal ganglia consist of a direct and an indirect pathway of motor control. In both pathways, the striatum is activated by the cortex, which forms a loop of control with the thalamus (the grey arrow). In the direct pathway (the solid black arrow), the activation of the striatum inhibits the activity of the internal segment of the globus pallidus (GPi) and the substantia nigra (SNr), which further inhibits thalamic functions. Therefore, cortical activation is associated with an increased thalamic activity via the direct pathway. In the indirect pathway (the black dashed line), the activation of the striatum inhibits the activity of the external segment of the globus pallidus (GPe), which further inhibits the activity of the subthalamic nucleus (STN). Notably, the activity of the STN further activates GPi/SNr, which decreases thalamic activity. Therefore, cortical activation is associated with a decrease in thalamic activity via the indirect pathway. (b) The cerebellum plays a key role as an internal model of motor learning. A forward model predicts the sensory outcomes when motor commands are executed. It adjusts sensorimotor processing via feedback of the predicted sensory outcomes. An inverse model calculates the motor commands that would produce the sensory outcomes from desired actions. According to Wolpert et al. (2001), both models are crucial to motor control.
4.1.3.5 Cerebellum
The cerebellum has long been conceived as a critical component of the motor system. Activation of the cerebellum is consistently found during chewing and drawing/writing (Yuan and Brown 2015; Lin 2018) together with other brain regions such as the M1 and the SMA. Activation of the cerebellum can also be identified during motor imagery tasks (Filgueiras et al. 2018) (Table 4.1). In addition to controlling a movement, the cerebellum plays multiple roles in planning and learning a movement. One of the key roles of the cerebellum is to control movement by integrating sensory and motor information. The importance of the cerebellum in motor learning can be exemplified by its role in the development of automaticity, which means the movement can be performed with ‘a decreased need for attention and cognitive control’ (Cohen and Poldrack 2008). For example, individuals do not need to pay much attention to their jaw movement during eating. When one is repeating a movement, an internal model was established for predicting the outcome of motor output (Wolpert et al. 1998). The development of automaticity of movement can be conceived as a process of prediction and error correction. In motor learning, individuals established an internal model that links sensory and motor information. A forward model predicts the sensory outcomes when motor commands are executed. In contrast, an inverse model calculates the motor commands that would produce the sensory outcomes from desired actions (Wolpert et al. 2001) (Figure 4.2b). The forward model adjusts sensorimotor processing via feedback of predicted sensory outcomes. In contrast, the inverse model generates motor commands in a feedforward direction (i.e. to achieve a movement). The cerebellum may play a key role in the forward and inverse models of motor control. It transforms the desired movement trajectory to the motor commands required for achieving the goal of movement (Wolpert et al. 1998) (Figure 4.2b).
While the cerebellum is conceived as a calculator that estimates the association between sensory feedback and motor command, it does not mean the cerebellum only works for sensorimotor learning. On the contrary, cumulating neuroimaging evidence has suggested that the cerebellum plays a key role in cognitive processing, evidenced by its intense connections with the associate cortex (Stoodley 2012). The cerebellum forms connections with multiple cortical regions, including the prefrontal, temporal and parietal lobes. The analysis of intrinsic functional connectivity (see Section 2.4) revealed that cerebellar lobule VI is associated with the salience network. Cerebellar crus I and crus II are associated with executive control and the lobule IX is associated with the default mode network (Sokolov et al. 2017). The intense connections between the cerebellum and the networks of cognitive processing may explain why cerebellar deficits are associated with a diverse of cognitive or emotional dysfunctions in addition to motor dysfunctions (Schmahmann et al. 2019).
4.1.4 Brainstem
The brainstem plays a key role in motor control of rhythmic movements, such as swallowing, chewing and breathing (Marder and Bucher 2001). The rhythmic pattern of oral functions is produced and maintained by central pattern generators (CPGs). A CPG refers to ‘a small network of neurons, or even a single neuron, whose activity can generate specific movements with correct timing and sequences in the absence of sensory feedback’ (Moore et al. 2014). A distinct feature of CPGs is that they can generate and maintain rhythmic activity independent of the input of timing information from an extrinsic source (Marder and Bucher 2001). Notably, the CPGs do not work standalone to maintain the pattern of mastication. The inputs from the brain and the peripheral sensory receptors, including periodontal mechanoreceptors and muscle spindles, also modify the pattern of masticatory movement via the CPGs (Lund and Kolta 2006). Most of our knowledge of CPGs has been based on animal research, and findings are summarized in previous reviews (Marder and Bucher 2001; Moore et al. 2014). Until now, there is a lack of a practical neuroimaging method for investigating the activity of human CPGs associated with oral functions, and the cross‐talk between CPGs and other cortical/subcortical regions has not been fully elucidated. The use of ultrahigh‐field magnetic resonance imaging (MRI) may also improve the imaging results of the brainstem by enhancing the spatial resolution of the images (Sclocco et al. 2018).
4.1.5 Summary
- The relationship between a motor programme and motor learning is dynamic. A motor programme can be adjusted by receiving feedback according to the outcome of the activities.
- Recent neuroimaging findings suggest that the M1 plays a predominant role in motor execution but is not limited to execution per se. The M1 works closely with the SMA/the PMC during the preparatory stage or merely by imagining a movement.
- In contrast to the M1, the SMA and the PMC, especially their rostral subregions, are more relevant to the attention and cognitive processing of a movement. The PMC is critical to integrate sensory information (which is usually an external guide of movements), and the SMA is critical to self‐initiate movement and maintaining learned movement sequences.
- The cerebellum plays a key role in tuning the sensory and motor information for motor control so that a motor command can be continuously updated and the movement can be controlled for accuracy and speed. The cerebellum also forms connections with multiple cortical regions, including the prefrontal, temporal and parietal lobes, contributing to cognitive and emotional processing.
- The CPGs of the brainstem play a key role in maintaining the rhythmic pattern of movements. However, how the CPGs cross‐talk with the other brain regions, especially for the top‐down control from the cortical motor regions, has yet to be explored in neuroimaging research.
4.2 Brain Mechanisms of Human Mastication
4.2.1 Introduction
For the past 20 years, neuroimaging has made a substantial contribution to our understanding of brain mechanisms related to human mastication. The neuroimaging findings are complementary to the findings from earlier clinical and animal research, which have extensively investigated the neurophysiological mechanisms of mastication. In this chapter, we do not focus on the general neurophysiological mechanisms of mastication. In contrast, we highlight the novel evidence provided by neuroimaging. We primarily focus on the findings and methodological issues of fMRI research on chewing movement and the findings of magnetoencephalography (MEG) and functional near‐infrared spectroscopy (fNIRS). Finally, a tentative model of the human masticatory process is proposed, based on the novel findings from neuroimaging research.
4.2.2 Earlier Findings from Animal Research on Chewing Movement
Before the advancement of neuroimaging, the findings from animal research have contributed to a general framework of the neurophysiological mechanisms of mastication (Kawamura 1964). A critical advantage of animal research is that, via direct intervention on the experimental animals, one can obtain more valid conclusions regarding the cause–effect relationship between structure and function. For example, the role of the motor cortex in maintaining oral functions can be demonstrated by the findings that in awake monkeys jaw and tongue muscle activities were disrupted when the face motor cortex was inactivated (Yamamura et al. 2002). Moreover, using animal models, researchers can explore such a relationship at the single‐neuron level (Lin and Sessle 1994; Lin et al. 1994). Several landmark findings have been demonstrated via animal research, including identifying the role of the cortical masticatory area (CMA) and the CPGs in jaw movement, and the plasticity of the sensorimotor circuitry of mastication, as outlined in the following sections (for detailed reviews, see Avivi‐Arber and Sessle (2018) and Lund (1991)).
4.2.2.1 Identification of the Role of the Cortical Masticatory Area
In rats and monkeys, the CMA has been identified as the cortical areas that generate rhythmic jaw movement by receiving repetitive stimulation (Isogai et al. 2012). The causal role of the CMA in normal mastication has been identified by animal research. For example, stimulating the anteromedial half of the cerebral cortex using prolonged electrical pulses triggered rhythmical masticatory movements in rabbits (Lund et al. 1984), and lesioning the masticatory area induced deficits of normal mastication in cats (Hiraba and Sato 2005). In addition, the CMA works with the putamen, one of the core components of basal ganglia, to form the corticostriatal pathway for jaw movement (Masuda et al. 2005). The CMA that induces chewing‐like rhythmic jaw movements also shows a strong connection with the thalamus (Isogai et al. 2012). Finally, recent evidence revealed that during development a lack of occlusal loading is associated with deficits of rhythmic jaw movements induced by the stimulation of the CMA (Aung et al. 2020). These findings further confirmed the key role of the CMA in the brain mechanisms of mastication.
4.2.2.2 The Sensorimotor Circuitry of Mastication and Its Plasticity
During mastication, the pressure and nociceptive receptors from the periodontium and the oral cavity, as well as the muscle spindles of the masticatory muscles, are the major sources of sensory feedback (Trulsson 2006). Animal research has provided much evidence regarding the integration of sensory and motor information related to mastication (Lin et al. 1994; Lin and Sessle 1994). Moreover, new evidence supports the notion that at the cortical level the sensorimotor circuitry may be sculpted in accordance with long‐term experience. For example, in rats, the modification of the pattern of occlusion (by trimming the incisor) was associated with an altered brain representation of the jaw and the tongue in the face region of the M1 (Avivi‐Arber et al. 2015). In mice, tooth loss (by extraction) induced a change in brain volume in widespread regions of the brain (Avivi‐Arber et al. 2017). The animal findings demonstrate the association between altered oral conditions and brain plasticity, which may relate to the rehabilitation of oral functions (Sessle 2019).
4.2.3 Experimental Design of Neuroimaging of Mastication
In the following sections, we outline the neuroimaging findings of human mastication, mainly based on fMRI research. A typical task‐based fMRI study of mastication consists of a task condition, in which subjects perform a chewing task, and a baseline condition, in which subjects do not chew but just rest. During the chewing task, subjects chew an experimental material, which is usually a piece of odourless chewing gum, for a fixed number of strokes. In order to identify the brain activation associated with chewing, the sessions of the chewing task were contrasted with the baseline (resting) sessions, in which chewing was not performed (Figure 4.3a). For example, in the study by Onozuka et al. (2002), subjects chewed a piece of gum for four cycles, 32 seconds for each cycle, during an fMRI scan. The blood‐oxygen‐level‐dependent (BOLD) signals from this task condition were contrasted to the signals from the baseline condition, i.e. four sessions of resting. Increased activation in the task condition compared to the baseline condition is considered to be associated with mastication (Onozuka et al. 2002) (Figure 4.3b).

Figure 4.3 Experimental design for neuroimaging of the brain mechanisms of chewing. (a) The basic concept of study design. The study consists of multiple blocks of a chewing task and a baseline (no‐chewing) block. (b) Variations of the study design. Different studies may differ in the number of blocks of tasks and the definition of the baseline block (e.g. resting or clenching the teeth). The variations lead to a different interpretation of imaging results.
The experimental design by Onozuka et al. (2002) helps to clarify the brain activation associated with mastication by contrasting the chewing conditions to non‐chewing conditions. However, the design cannot disclose the brain activation associated with a specific stage during mastication. Mastication may consist of different stages, and sensorimotor processing may vary between the stages. For example, individuals may perceive stronger sensory feedback when they start to chew a hard material and adjust to a rhythmic pattern of chewing when they get used to the material. Finally, they need to stop the rhythmic movement and prepare for swallowing. In order to investigate the brain mechanisms of different stages of mastication, Quintero et al. adopted a design similar to the one used by Onozuka et al. (2002). In addition, they divided the chewing session evenly into five segments or ‘time windows’ (Figure 4.3b) (Quintero et al. 2013a). By comparing the brain activation between these segments, they were able to delineate the brain activation associated with a specific stage of mastication, such as the period when chewing was just initiated and the period when chewing was terminated.
As discussed in Section 2.1, all the neuroimaging evidence needs to be evaluated for its internal and external validity. In the neuroimaging research on mastication, the lack of internal validity means that the observed brain activation may not truly reflect the masticatory process. For example, in most neuroimaging studies, subjects chew a piece of odourless chewing gum. If the chewing gum has some flavour, the brain activation may reflect the gustation related to the taste of the gum rather than mastication. Therefore, the factors (e.g. the taste of the gum) that may confound the desired function (e.g. mastication) should be well controlled in experimental design. Nevertheless, when the experimental condition is well controlled for every aspect, the result derived from the study may not provide good external validity. In other words, the conclusion from the research cannot be generalized in our daily life. For example, researchers may ask all the subjects to chew a piece of odourless gum for 10 cycles, with the frequency of one second per cycle, so that the conditions of the task can be standardized across all subjects. However, the ‘mastication’ performed during the task is far different from that we have performed in our daily life. In other words, the task condition – which is highly constrained by many factors – does not really reflect how individuals would really chew during eating. These issues of external validity should be carefully explained when it comes to a study of elderly and special needs patients. In daily life, these patients may face many difficulties of feeding (e.g. having the risk of being choked), which are hard to be ‘simulated’ in a task condition during an imaging scan.
Table 4.2 Neuroimaging research on the brain mechanisms of mastication (since 2015).
Source | Participants | Methods |
---|---|---|
Non‐treatment‐related research | ||
Lin et al. (2020b) | 31 patients of cognitive impairment (31 healthy controls) | sMRI/VBM |
Lin et al. (2020a) | 40 patients of cognitive impairment (30 healthy controls) | sMRI/VBM |
Nagashima et al. (2020) | 35 healthy adults | fNIRS/mastication and foot stepping |
Sörös et al. (2020) | 17 healthy adults | fMRI/frontal, horizontal and vertical tongue movements |
Feng et al. (2019) | 10 healthy adults | fMRI/occlusion of the left first premolar, left second premolar and right first premolar |
Yoshizawa et al. (2019) | 15 healthy adults | fMRI, EMG/incisal and molar biting at three step‐wise force levels |
Lin et al. (2017) | 26 older and 26 younger healthy adults | rs‐fMRI/graph‐based network analysis |
Kawakami et al. (2017) | 14 healthy adults | fNIRS/gum chewing and a computerized Stroop test |
Choi et al. (2017) | 8 healthy adults | fMRI/gum chewing |
Lotze et al. (2017) | 15 healthy adults | fMRI/chewing on a unilateral side |
Viggiano et al. (2015) | 18 healthy adults | ASL‐MRI/gum chewing on a unilateral side |
Jang et al. (2015) | 9 healthy adults | fMRI/hand movements w/wo gum chewing |
Sakamoto et al. (2015) | 13 healthy adults | EEG/a somatosensory Go/no‐go task w/wo gum chewing |
Jiang et al. (2015) | 16 healthy adults with a right or left chewing‐side preference | fMRI/rhythmic chewing |
Lin et al. (2015) | 25 older adults | sMRI, rs‐fMRI/VBM and functional connectivity |
Treatment/disease‐related research | ||
Kanzaki et al. (2019) | 25 patients with mandibular prognathism (17 subjects with normal occlusion) | fNIRS, EMG/a calculation task and a chewing task |
Narita et al. (2019a) | 16 partially edentulous patients | fNIRS, EMG/chewing w/wo wearing a denture |
Ozdiler et al. (2019) | 16 patients of Class II malocclusion, 8 wearing appliances full time and 8 wearing appliances at night while sleeping (10 pre‐treatment controls) | fMRI/chewing and biting movements |
Narita et al. (2019b) | 15 patients with occlusal dysesthesia (15 healthy controls) | fNIRS/chewing |
Inamochi et al. (2017) | 28 fully dentate adults wearing experimental denture‐base palatal plates | fMRI on pre‐insertion (Day 0), Day 1, Day 3 and Day 7 after insertion/chewing |
Fan et al. (2017) | 12 possible sleep bruxism patients (12 healthy controls) | 1H‐MRS |
Kamiya et al. (2016) | 12 elderly edentulous subjects (12 young healthy controls) | fNIRS and EMG/chewing w/wo wearing a denture |
Perumal et al. (2016) | 20 edentulous patients wearing complete dentures | EEG/before and after chewing and w/wo wearing a denture |
Tramonti Fantozzi et al. (2019) | 8 patients with clicking sounds | fMRI, EMG/a finger to thumb motor task with teeth in direct contact or with a bite interposed between arches |
Banu et al. (2016) | 10 edentulous patients receiving an implant‐supported overdenture | EEG on six different phases of assessment |
Yılmaz (2015) | 12 patients with bruxism (12 healthy controls) | fMRI/tooth clenching |
Notes: ASL‐MRI: arterial spin labelling magnetic resonance imaging; EEG: electroencephalography; EMG: electromyography; fMRI: functional magnetic resonance imaging; fNIRS: functional near‐infrared spectroscopy; MRS: magnetic resonance spectroscopy; rs‐fMRI: resting‐state functional magnetic resonance imaging; sMRI: structural magnetic resonance imaging; VBM: voxel‐based morphometry.
4.2.4 Neuroimaging Findings of Brain Mechanisms of Mastication
In the next sections, we briefly review the recent finding of the brain mechanisms of mastication from neuroimaging research (Table 4.2). In these sections, we primarily focus on the findings from fMRI published in recent years. Key findings from other imaging modalities, such as MEG and fNIRS, are discussed.
4.2.4.1 The Sensorimotor Circuitry of Mastication
A recent meta‐analysis derived from the results of 20 fMRI studies (including 13 studies of mastication and 7 studies of clenching) identified the brain regions most consistently associated with mastication (Lin 2018). These regions include the bilateral M1, the right S1, the bilateral thalamus, the SMA and the cerebellum (Lin 2018) (Figure 4.4). Both chewing and clenching are associated with consistent activation at the right M1. Chewing is additionally associated with brain activation at the left M1, compared with ‘clenching’ (i.e. a condition without rhythmic jaw movement). Across the 13 studies of chewing movement, the loci of brain activation showed great inter‐study variations. Activation at the M1 and the PFC has been consistently identified in most of the studies (n = 10 and n = 9, respectively). Activation at the S1, the SMA, the thalamus, the insula and the cerebellum was also reported (n = 6). The findings revealed that the primary sensorimotor area is critically associated with both chewing and clenching. Moreover, in addition to the M1 and S1, the secondary motor area (including the SMA and the PMC), the PFC and the cerebellum also contribute to the brain mechanisms of mastication. The meta‐analytical results generally confirmed the findings from animal research. Moreover, based on the meta‐analytical results, the loci of activation at the S1 and M1 are consistent with the somatotopic region of the orofacial area, i.e. the lateral and ventral areas of the M1 and the S1. As a critical component of the descending motor system, the thalamus showed reciprocal connections with the CMA for rhythmical jaw movements (Isogai et al. 2012). Consistently, the meta‐analysis identified consistent brain activation at the thalamus among the studies of chewing movement (Lin 2018). Hemispheric dominance was frequently found in the sensory and motor cortices for limbs. In a recent arterial spin labelling magnetic resonance imaging (ASL‐MRI) study, Viggiano et al. (2015) found increased blood perfusion in the right trigeminal principal nucleus after subjects conducted a mastication exercise, regardless of the side of chewing. For jaw movement, an electroencephalography (EEG) study revealed that during mouth opening and closing, the movement‐related cortical potentials were symmetrically distributed (Yoshida et al. 2003). An fMRI study also showed bilateral activation during chewing (Lotze et al. 2017). The bilateral activation at both hemispheres was also identified in the meta‐analytic results (Lin 2018).

Figure 4.4 Brain activation associated with chewing and clenching.
Source: Lin (2018). Reproduced with permission of John Wiley and Sons.
In addition to fMRI, MEG and fNIRS have also provided critical evidence regarding the brain mechanisms of mastication. Using MEG combined with a jaw‐motion tracking device, researchers have recorded the brain activity associated with the jaw movement of mouth opening and phoneme production (i.e. to speak a/pa/sound). The findings revealed the role of the M1 in the jaw movement for both chewing and speech (Memarian et al. 2012). In addition to jaw movement, tongue movement also plays a key role in mastication. One study investigated the MEG and electromyographic (EMG) signals during tongue protrusion and found a MEG–EMG coherence at the bilateral hemispheres, regardless of the side of tongue protrusion (Maezawa et al. 2014). An earlier fNIRS study showed a consistent activation at the somatosensory cortex when subjects received painless vibrotactile stimuli, regardless of the position of the teeth (maxillary or mandibular incisors, canines, first premolars and first molars). Moreover, the first molars showed stronger activation compared to the other teeth (Shimazaki et al. 2012). Another study investigated the effect of different intensities during clenching, which was calibrated to 20%, 50% and 80% of the maximum clenching force. The results showed an increased fNIRS activity in the primary sensory and motor cortices between the different levels of intensity (Shibusawa et al. 2009). Consistently, recent fMRI studies reveal an extensive activation in the primary sensorimotor area during occlusion of a single premolar (Feng et al. 2019). Stronger activation in the primary sensorimotor area and the cerebellum was associated with greater strength during a molar biting task (Yoshizawa et al. 2019). Together, the results highlight the role of the primary sensorimotor area in occlusion.
4.2.4.2 The Secondary Motor Area
The secondary motor area consists of the PMC and the SMA (Nachev et al. 2008; Picard and Strick 2001). As shown in Section 4.1, both regions are critical to motor control, especially for planning and learning a movement. The PMC was frequently reported in the learning task when the learners are required to develop a movement guided by an external cue. In contrast, the SMA was found when the learner has internalized the motor plan after repeated practice. In this condition, the learner will develop a movement mainly guided by his/her memory (Debaere et al. 2003). Because most of the fMRI studies focused on the session when subjects were executing the chewing movement (Table 4.2), it is not surprising that activation at the M1 was reported more frequently compared to the PMC or the SMA. The activation of the SMA has been identified in earlier research (Onozuka et al. 2002) and it is more frequently reported compared to the PMC, according to the meta‐analytic results (Lin 2018). The PMC activation is often associated with a movement guided by an external cue, such as visual feedback (Debaere et al. 2003). Therefore, the lack of PMC activation may reflect the nature of the intraoral movement, which is less dependent on the guidance from an external cue. A recent study on structural features of older people revealed that grey matter volume at the motor regions, including the M1 and the PMC, was associated with the individual masticatory performance (Lin et al. 2015). Since the structural features of the brain reflect the intrinsic architecture of the brain, the findings suggested that structural variability in the motor regions may be associated with the individual difference in masticatory function.
4.2.4.3 The Prefrontal Cortex
One of the notable findings from the recent neuroimaging studies is the activation of the PFC during chewing. As discussed earlier, prefrontal activation has been seen in many fMRI studies of chewing movement (Lin 2018). However, there exist great inter‐study variability in the loci of activation, which were widely distributed in the whole frontal area (Lin 2018). Activation at the PFC has been identified since the early days of neuroimaging research on chewing (Onozuka et al. 2003). Onozuka et al. (2003) found higher activation in the PFC during a chewing task in the older subjects compared to the younger subjects. The PFC activation was not pronounced for younger subjects (Onozuka et al. 2002; Quintero et al. 2013a). Brain activation at the frontal and parietal lobes differed between tongue movements in different directions (Sörös et al. 2020). Activation of the frontal and parietal lobes was also associated with chewing‐side preference (Jiang et al. 2015). When mastication was conducted at the same time as other ongoing tasks (e.g. hand movement and a decision task), changes in brain activity were found (Jang et al. 2015; Sakamoto et al. 2015). The findings suggest that the PFC may not play a consistent role in the sensorimotor circuitry of mastication, such as the M1, the SMA and the cerebellum. Instead, the PFC activation may signify the individual and contextual factors related to mastication, such as subjects’ age and the attention and cognitive processing during the chewing task.
The role of the PFC activation has also been identified in neuroimaging studies of dental patients (Table 4.2). To study jaw movement related to oral rehabilitation (e.g. using a denture), fNIRS would be a suitable tool because subjects can perform a chewing task under less physical restriction. In contrast, in fMRI studies, subjects’ movement can be constrained inside an MRI scanner. Using fNIRS, Narita et al. (2019a) found an increased prefrontal activation when edentulous subjects chewed with a denture compared to chewing without wearing a denture. Kamiya et al. (2016) report that when the elderly edentulous subjects chewed with their dentures, the prefrontal activation was not significantly different from that in the young participants. In another study, Kanzaki et al. (2019) compared the brain blood flow during chewing between subjects of normal occlusion and mandibular prognathism. They found increased activity in the inferior frontal gyrus in both groups, but the increase was smaller in the patients with mandibular prognathism (Kanzaki et al. 2019). The findings suggest that the PFC activity may reflect the individual difference in age, the experience of wearing a denture or the pattern of occlusion. Furthermore, the PFC activity may be associated with the inter‐individual variability in attention and cognitive processing of mastication. For example, in older subjects, mastication may improve subjects’ generalized attention, which may be associated with a greater PFC activity (Nagashima et al. 2020). Chewing was also associated with increased activity of the dorsolateral PFC when subjects were performing a Stroop test, which was engaged with attentional processing (Kawakami et al. 2017). PFC activity may also reflect the individual difference in perceptual processing of chewing. For example, a recent fNIRS study revealed that the patients with occlusal dysesthesia showed a lower PFC activity during chewing compared to healthy controls. Moreover, in the patients, the PFC activity was associated with the severity of somatization, assessed by the somatization subscales of the Symptom Checklist‐90‐R (Narita et al. 2019b). In a longitudinal fMRI investigation on healthy subjects wearing a palatal plate, Inamochi et al. (2017) found decreased activation at the superior frontal gyrus at the time when the subjects have worn the plate for seven days compared to the time right before the plate was inserted. The accumulating evidence further suggests that the PFC may play a key role in the adaptation of oral conditions (Lin 2019).
4.2.4.4 Cerebellum
In addition to the M1/S1 and the SMA, the cerebellum is another brain region commonly identified in fMRI studies of chewing movement (Lin 2018). However, because the cerebellum consists of structurally and functionally heterogeneous subregions (see Section 4.1), it would be critical to differentiate the subregions that were associated with chewing movement. According to the meta‐analytic results, the bilateral lobule VI and crus I are consistently identified in the fMRI studies (Lin 2018). Notably, both the lobule VI and the cerebellar crus are part of the posterior lobe of the cerebellum, which may play a key role in cognitive processing, as demonstrated by its connectivity with the associative cortices and its association with cognitive impairment (Stoodley and Schmahmann 2010).
One hypothesis of the role of cerebellar activation is the development and maintenance of the ‘automaticity’ of chewing movement. In terms of motor control, automaticity generally refers to ‘a decreased need for attention and cognitive control’ associated with cognitive or motor tasks (Cohen and Poldrack 2008). Moreover, developing and maintaining the automaticity of motor control is associated with extensive practice, which can be conceived as a process of refining the model of motor control by sensory and motor information (Schmahmann et al. 2019; Cohen and Poldrack 2008). If the cerebellum is critical to the automaticity of motor control, one may conceive that a functional connection exists between the cerebellum and the sensorimotor cortices. This is supported by the findings from task‐based fMRI research. During chewing, there was a positive correlation of the signals between the posterior lobe of the cerebellum and the M1 (Quintero et al. 2013b). In addition, findings from structural magnetic resonance imaging (sMRI) research revealed that older people, those who showed a higher masticatory performance, showed a stronger resting‐state functional connectivity between the PMC and the cerebellum (Lin et al. 2015). These findings suggest that the individual variability in the architecture brain connectivity may be associated with their oral functions.
4.2.5 Synthesis of the Neuroimaging Evidence of Mastication
So far, we have reviewed the new evidence from neuroimaging that adds to our current knowledge of the mechanisms of human mastication. In the following section, we first focus on the major challenge of neuroimaging research on mastication, i.e. a lack of cause–effect mechanisms about human mastication, and an integrated framework of human mastication, focusing on the ‘masticatory process,’ is proposed.
4.2.5.1 Investigating Cause–Effect Mechanisms of Mastication
Animal studies have identified the role of the CMA and the CPGs in controlling rhythmic jaw movements. Researchers found that the pattern of jaw movement can be modified via stimulating the CMA and the CPGs in animal subjects. The findings support a causal role of the CMA and the CPGs in maintaining jaw movements (Lund 1991; Nakamura and Katakura 1995). Neuroimaging research provides evidence from directly assessing human subjects. However, most neuroimaging evidence only accounts for the correlation, but not the cause–effect relationship, between the brain and behaviour (see Box 4.2 and Box 4.3 in the Companion Website). The cause–effect mechanisms of the brain can be elucidated by adopting new technology and experimental design. Firstly, the causal effect can be better clarified by directly manipulating the activity of brain regions and observing how the relevant behaviour is altered. This can be performed by using interventional approaches of neuroimaging, such as transcranial magnetic stimulation (TMS) or transcranial direct current stimulation (TDCS). Both TMS and TDCS belong to the approaches of brain stimulation, which alter brain activity in a non‐invasive way. At present, the use of interventional approaches has been rarely seen for modifying mastication (but more frequently for modifying swallowing, see Section 4.3). Secondly, one may consider improving the research design of neuroimaging studies. At present, most of the studies have been cross‐sectional research (Table 4.2). Longitudinal research with a prospective design may better capture the effect of different factors on mastication. For example, the study by Inamochi et al. (2017) traced both brain activation and masticatory performance for seven days. They found that the brain activation at the S1 and the putamen presents a similar pattern as the improvement of masticatory performance after installing a denture (Inamochi et al. 2017). An earlier study also identified a temporal change of brain activation after denture installation, but the activation returned to its original level in the long run (Luraschi et al. 2013). The understanding of the cause–effect relationship plays a pivotal role in clinical applications because it provides a more precise mechanism for treatment planning and predicting treatment outcomes.

VIDEdental - Online dental courses
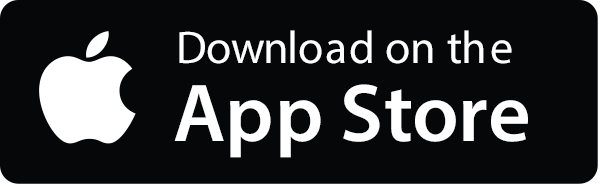
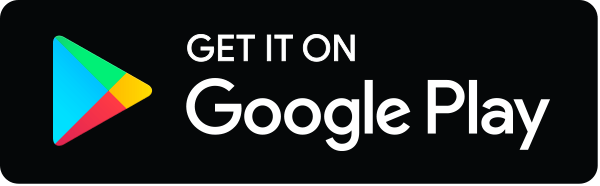