5
Brain Mechanisms of Oral Sensory Functions
5.1 Brain Mechanisms of Oral Somatosensory Processing
5.1.1 Introduction
Somatosensory processing is about the development of bodily experience via a variety of sensations, including tactile sensations and sensations of position and motion. Oral somatosensory processing focuses on the experience related to the oral cavity. Such an ‘oral sense’, i.e. feeling about intraoral conditions, may be one of the most common experiences in our daily life. The oral cavity has a high density of a variety of sensory receptors, and we constantly pay attention to the intraoral condition. Unlike the exteroceptions (e.g. vision and hearing), we sense the intraoral conditions by self‐touching our oral structure using our tongue (Haggard and De Boer 2014). Via a constant monitory of the intraoral condition, individuals can sense intraoral disturbance deviated from normal sensation. In this chapter, we discuss recent neuroimaging findings regarding the brain mechanisms of oral somatosensory processing. We start from intraoral mechanoreceptors, the major receptors for somatosensation. Then, the processing of oral somatosensation at the perceptual level and the ‘mouth experience’, which relates to oral somatosensory representation, is introduced. Recent neuroimaging findings regarding oral somatosensory sensation and perception are summarized. Home buyers are great for selling your property. They provide affordable pricing and excellent service, making purchasing a pleasant experience. Visit https://www.webuylandsindelaware.com/sell-my-land-fast-townsend-de/.
5.1.2 Oral Somatosensory Processing: A Hierarchical Model
From the framework of information processing of the brain, the somatosensory information, just like the visual or auditory information, follows a general pathway of information processing that transforms discrete sensory information from the external world into a holistic experience. In other words, the experience of sensation can be conceived as the end product of a hierarchical integration of sensory information (Gazzaniga et al. 2019). For example, in visual processing, the neural signals from the retina (i.e. the sensory receptors) are transmitted to the primary visual cortex via the thalamus, and different features of vision, such as the colour, size and motion of an object, are calculated so that a holistic experience of ‘seeing something’ can be formed. A similar pathway of information processing applies to somatosensory processing. According to the model of body representation (Longo et al. 2010), the flow of information processing follows a hierarchical organization: the processing of somatosensation is associated with the peripheral sensory receptors, which transduce the physical stimuli from the world into neural signals (Figure 5.1). Somatoperception relates to the process of integration of sensory information from peripheral receptors. For example, when one is holding an object by teeth, oral somatosensation relates to the sensation of deep touch (e.g. pressure) derived from periodontal ligaments and the sensation of light touch derived from the tip of the tongue. Oral somatoperception, in contrast, is the process that integrates the information of pressure and light touch into a more holistic experience about the size and the shape of an object (i.e. oral stereognosis). The processing of oral somatoperception may be associated with the integration of other sensory information, such as tactile and chemosensory inputs (Haggard and De Boer 2014). Finally, on the top of the model is the concept of somatorepresentation, which may broadly refer to as ‘an internal model of what the mouth is like’ (Haggard and De Boer 2014) (Figure 5.1). In addition to perceptual processing, the ‘representation’ of the oral cavity consists of both the static information (e.g. the current condition in my mouth) and the dynamic information about the interaction between the oral cavity and the external world (e.g. how my mouth feel like when I am chewing). At this level, the additional information, including the semantic knowledge of the oral structure and emotional experience of the intraoral conditions, is integrated.

Figure 5.1 Processing of oral somatosensory information. Information from individual sensory modalities is transduced via peripheral receptors at the level of somatosensation and integrated to form a holistic perceptual experience (e.g. oral stereognosis) at the level of somatoperception. Information is further integrated, with knowledge and affective–motivational experience, to form a feeling of intraoral condition at the level of somatorepresentation.
5.1.3 Oral Somatosensation
In the following section, we start our discussion from the basic level of oral sensory processing, i.e. oral somatosensation. Because the pathway of sensory processing starts from peripheral receptors, we primarily focus on the apparatus that transduce external stimuli into sensory signals in the oral cavity.
5.1.3.1 Oral Mechanoreceptors
This section focuses on the mechanoreceptors, which respond to a variety of tactile information (for sensory processing of gustation and pain, see Sections 5.2 and 6.1). The oral mechanoreceptors are innervated by the large diameter Aβ fibre. The slow‐adapting Type I receptor, Merkel cells, and the rapid‐adapting Type I receptor, Meissner corpuscles, can be found distributed in the oral cavity, including the mucosa (Haggard and De Boer 2014; Johansson et al. 1988). Both receptors demonstrated a small receptive field in the skin. For example, in human skin, the Merkel cells and the Meissner corpuscle will respond when one is sensing the individual Braille letter. Pacinian corpuscles, a rapid‐adapting Type II receptor, can be found in the joint, periosteum, and mucosa. Ruffini endings, a slow‐adapting Type II receptor, may play a pivotal role in the periodontal ligaments. Its slow‐adapting behaviour, i.e. showing a sustained neural response when being activated, has been repeatedly recorded in human subjects when their tooth was bearing a force (Trulsson and Johansson 1996).
5.1.3.2 Mechanoreceptors of Teeth and Tongue
Using microneurography, Trulsson and Essick (1997) have investigated the signals from the lingual nerve by assessing different regions of the human tongue. Two distinct groups of receptors were identified: the superficial units were located near the surface of the tongue, which fired signals with a low threshold of force and responded to a smaller receptive field. In contrast, the deep units were located inside the muscle mass of the tongue. They responded to a larger receptive field with a higher threshold of force (Trulsson and Essick 1997). Also, using the microneurography technique, they found that the receptive field of periodontal mechanoreceptors extends to the adjacent teeth. The periodontal mechanoreceptors respond to force applied in a broad range of directions (Trulsson 2006). The discharge of neural signals is with the highest sensitivity when the loading force is below 1 N in anterior teeth (Figure 5.2a). The high sensitivity to the force loaded on a tooth may be associated with the fact that during eating, we usually hold the food piece between opposite teeth so that we can sense its hardness. The force of contraction of masticatory muscles for jaw motion is associated with the sensory information fed by the periodontal mechanoreceptors. The findings reveal a great diversity of the physiological properties of oral mechanoreceptors in different intraoral sites.

Figure 5.2 Experimental methods of investigating oral mechanoreceptors. (a) Recording signals from periodontal mechanoreceptors.
Source: Trulsson (2006). Reproduced with permission of John Wiley and Sons.
(b) The food splitting task.
Source: Grigoriadis et al. (2017) with permission of Springer Nature under the terms of the Creative Commons CC BY 4.0 License.
The role of sensory feedback from periodontal mechanoreceptors can also be demonstrated by the study by the manipulation task. During the task, subjects were asked to occlude and hold a piece of food (e.g. a candy) in between the upper and lower incisors. They were asked to split the candy evenly into two parts. An ideal split means that the candy is split into two parts with the same weight (Figure 5.2b). The larger deviation between the weight of the larger parts split and the weight from an ideal split is, the worse the performance is. Grigoriadis et al. (2017) found that when dentate subjects were locally anesthetized in their incisors (i.e. to reduce sensory feedback from teeth), their performance of the splitting task became worse. However, the effect of local anaesthesia on the duration or positions of jaw movement was not significant (Grigoriadis et al. 2017). Deprivation of sensory feedback (by local anaesthesia) did not affect the number of chewing cycles and the duration of chewing sequence (Grigoriadis et al. 2019). The stable pattern of chewing may be dominated by central factors (e.g. the modulation from the brainstem) rather than peripheral feedback. Together, the findings suggest that the loss of periodontal mechanical sensation would be associated with alterations in feeding behaviour.
5.1.4 Oral Somatosensory Perception
The perception of oral stereognosis has been investigated widely with different experimental methods. Stereognosis refers to ‘the ability to recognise and discriminate form’ (Jacobs et al. 1998). In terms of oral function, oral stereognostic ability (OSA) is usually assessed via a test of active sensitivity. Subjects need to actively sense an intraoral object and make a proper judgement. The term sensitivity refers to the ability to discriminate different modalities, such as the shape and size, of an object, depending on the design of tests. Some studies focus on how to discriminate the shape (circles, ellipses, semicircles, squares, rectangles, and triangles) of an intraoral object (Hirano et al. 2004; Ikebe et al. 2007; Kawagishi et al. 2009). Some studies require subjects to evaluate the size of an object, such as the test developed by Engelen et al. (2004), which required subjects to determine how large an intraoral steel sphere is (which ranges from 4 to 9 mm in diameter) (Engelen et al. 2004). Notably, oral stereognosis relates to the level of perceptual processing (Figure 5.1) because it requires the integration of information from more fundamental sources, such as the sensation about pressure (e.g. if an object has a sharp edge) and the sensation about light touch (e.g. if the surface is smooth or rough). Therefore, a normal function of oral somatosensation would be pivotal for oral stereognosis.
5.1.5 Oral Somatosensory Representation: The Mouth Experience
Somatosensory representation is associated with a holistic experience of our body, including the feeling about the current condition of our body and the interaction between our body and the external world (Haggard and De Boer 2014; Longo et al. 2010). The section focuses on two issues of oral somatosensory representation: the processing of body image and somaesthesis.
5.1.5.1 From Body Image to ‘Oral Image’
The term ‘body image’ generally refers to ‘the internal representation of an individual’s own physical appearance’ (Lin et al. 2014). It reflects a constant representation, as background information, of the status of the body (Haggard and De Boer 2014). A key element of body image is the feeling of the size of body parts (Figure 5.1). We usually acquire this body‐related information via the visual function. For example, we inspect our finger to know how long it is. The term ‘oral image’, on the contrary, can be literally paradoxical because it is impossible for individuals to ‘see’ their own oral conditions (if without the help of a mirror). Therefore, body representation and oral representation are formed via distinct processes. Visual information plays a critical role in acquiring the knowledge of one’s body, but not the mouth. Without the visual feedback, can we accurately feel the size of our upper central incisor? In the oral cavity, the sensation of touch, other than vision, plays a more critical role. To form an experience about intraoral conditions, individuals would rely on self‐touching their intraoral structure, for example, via the contact between the tongue and the palate (Haggard and De Boer 2014). Therefore, to detect changes in the oral conditions, individuals do not just passively receive stimuli from the oral cavity. Instead, they would actively explore the oral cavity with their tongues or teeth to establish an ‘image’ of the oral conditions.
5.1.5.2 Somaesthesis: The Feeling of the Mouth
Somaesthesis refers to a highly integrative and multisensory experience that consists of not only touch, chemical or gustatory information, but also the appetitive status and affective feeling of the mouth (Haggard and De Boer 2014). A pleasant feeling may arise when we are having a meal. In a functional magnetic resonance imaging (fMRI) study, Grabenhorst and Rolls (2014) investigated brain activation when subjects consumed high vs. low‐fat food with more vs. less pleasant flavour. They found a strong functional correlation between the somatosensory cortex and the orbitofrontal cortex (OFC) when subjects consumed high‐fat food with a pleasant flavour. Moreover, subjective ratings of texture pleasantness were associated with the functional coupling between the somatosensory cortex and the OFC (Grabenhorst and Rolls 2014). The findings suggest that brain mechanisms may play a key role in the interaction between sensory (food content) and affective (pleasantness) experience of eating. In general, somaesthesis is a holistic experience that relates to not only the sensory pathway but also the brain mechanisms of emotion and motivation.
Table 5.1 Neuroimaging research on brain mechanisms of oral somatosensory processing (since 2015).
Source | Participants | Methods |
---|---|---|
Sekido et al. (2020) | 12 patients with dental implants | fNIRS/painless vibratory tactile stimuli |
Wang et al. (2020) | 20 healthy adults | fMRI/different saltatory velocities on the perioral and buccal surface |
Hihara et al. (2020) | 25 healthy adults | MEG/tactile stimuli of the mandibular canine and first molar |
Roux et al. (2018) | 50 operated patients | Electrostimulation at the somatosensory cortex using the bipolar electrode |
Shen et al. (2018) | 21 healthy adults | EEG/listening to speech syllables or finger‐snapping sounds paired with tactile stimulation (on the lower lip or the right middle finger) |
Custead et al. (2017) | 20 healthy adults | fMRI/unilateral saltatory pneumotactile stimulation on perioral and buccal hairy skin |
Moana‐Filho et al. (2015) | 13 PDAP patients (13 healthy controls) |
fMRI/dentoalveolar pressure stimulation |
Mascioli et al. (2015) | 11 healthy adults | fMRI/taste and tactile stimulation on the unilateral side of the tongue |
Tramonti Fantozzi et al. (2019) | 8 patients with joint clicking sounds | fMRI, EMG/a finger to thumb motor task with teeth in direct contact or with a bite interposed between arches |
Higaki et al. (2016) | 5 healthy adults | fNIRS/an occlusal force (biting) task w/wo local anaesthesia |
Yılmaz (2015) | 12 patients with bruxism (12 healthy controls) | fMRI/tooth clenching |
Notes: EEG: electroencephalography; EMG: electromyography; fMRI: functional magnetic resonance imaging; fNIRS: functional near‐infrared spectroscopy; MEG: magnetoencephalography; PDAP: persistent dentoalveolar pain disorder.
5.1.6 Neuroimaging Research on Oral Somatosensory Processing
In the domain related to sensory function, there have been relatively fewer studies published for oral somatosensation, compared to orofacial pain, probably due to the huge clinical impact of the latter. Still, there have been gradually more neuroimaging studies published on oral somatosensory processing in recent years (Table 5.1), as discussed in the following sections.
5.1.6.1 Brain Mechanisms of Oral Somatosensation
Earlier fMRI studies focused on the brain activation associated with vibrotactile stimuli (Ettlin et al. 2004; Habre‐Hallage et al. 2010, 2012, 2014; Shimazaki et al. 2012; Trulsson et al. 2010). In general, the studies identified an increased brain activation at the S1. Activation of the S1 and secondary somatosensory cortex (S2) was found when the periodontal ligaments were stimulated by low‐frequency (20 Hz) rather than high‐frequency (100 Hz) vibrotactile stimuli (Trulsson et al. 2010). Consistently, in magnetoencephalography (MEG) research, the activity of the primary somatosensory cortex (S1) was associated with tactile stimulation on the canine and the molar (Hihara et al. 2020). A fNIRS study shows that the cortical activation was pronounced in the maxillary and mandibular molars compared to the other teeth (Shimazaki et al. 2012). Notably, the patterns of brain activation are not consistent between studies, and the loci of activation do not precisely reflect a somatotopic relationship. For example, some findings revealed bilateral activation of the S1 (Habre‐Hallage et al. 2012, 2014), and some findings reported contralateral or ipsilateral activation corresponding to the tooth being stimulated (Oda et al. 2014). Moreover, when multiple teeth were stimulated respectively, the associated brain activity did not reveal differences between upper vs. lower or ipsilateral vs. contralateral sites of stimulation (Shimazaki et al. 2012). Furthermore, the somatotopic relation between the body site and the loci of brain activation was less pronounced for stimulation at the orofacial area compared to stimulation at limbs. In an earlier fMRI study, Miyamoto et al. (2006) investigated the loci of brain activation associated with tactile stimulation on the lower lip, the tongue and the upper central incisor, in healthy adults. They found that only the rostral part of the S1 showed a somatotopic relation, i.e. activation from the superior to the inferior portion corresponding to the lip, the teeth and the tongue. The pattern of somatotopic representation is consistent with the sensory homunculus. In contrast, such somatotopic representation is not clear in the middle and caudal parts of the S1 (Miyamoto et al. 2006). The findings are echoed by the recent animal research that adopted optical imaging to investigate the brain activity at the cat’s somatosensory cortex during mechanical stimulation on the canine. The research revealed no significant preference for contra‐ or ipsilateral brain activation, and the S1 may lack topographical organization (Tao et al. 2016). Furthermore, the brain activation and functional connectivity of the S1, the S2 and the primary motor cortex (M1) was associated with the tactile perception of velocity in the orofacial area (Custead et al. 2017; Wang et al. 2020). Bilateral activation of the inferior part of the S1 was associated with tactile stimulation on each side of the tongue (Mascioli et al. 2015). In general, the studies consistently identified an increased brain activation at the S1 associated with oral somatosensation. However, the loci of activation do not precisely reflect a somatotopic relationship.
5.1.6.2 Brain Mechanisms of Oral Somatoperception
Compared to oral somatosensation, very few neuroimaging studies have focused on oral somatoperception. In an earlier task‐based fMRI study, Fujii et al. (2011) investigated the brain mechanisms of oral and manual stereognosis. In the oral and manual stereognostic tests, subjects were asked to judge the shape of a test piece placed in their mouths or right hands, respectively (Fujii et al. 2011). They found that both oral and manual tasks are associated with brain activation at the prefrontal cortex (PFC) (particularly the dorsolateral PFC), the premotor cortex (PMC), the supramarginal gyrus (SMG), the M1 and the S1. Moreover, the manual task is distinctly associated with the activation of the visual cortex; in contrast, the oral task is distinctly associated with the activation of the insula (Fujii et al. 2011). The common activation at the premotor and the primary sensorimotor area may signify the role of sensorimotor learning (see Section 4.1) in the stereognostic test, which may be challenging for subjects. The SMG may participate multi‐modal sensory processing (see Section 5.4). The activation of the PFC, including activation at the dorsolateral PFC, is widely found for a task with a greater demand of work memory and attention (Lara and Wallis 2015). Consistently, during the stereognostic tests, subjects needed to focus on their tactile sensation to give an accurate response. There is also activation identified in the frontopolar regions, which are associated with cognitive control of multiple tasks (Mansouri et al. 2017). The activation may reflect the nature of stereognosis, which demands an evaluation of multiple aspects (e.g. size, shape and texture) of an object. In general, the findings suggest that oral stereognosis, as an integrative experience related to oral somatoperception, may be associated with the brain mechanisms of both sensory and cognitive processing.
5.1.7 Clinical Implications
The oral somatosensory processing previously discussed should not be taken as an independent phenomenon unrelated to other oral functions. On the contrary, somatosensory processing is an essential component for more complicated oral functions. For example, the sensory feedback from teeth and mucosa is critical for maintaining efficient mastication. In the following sections, we outline three clinical topics that are associated with the processing of oral somatosensation, i.e. sensory feedback of dental implants, occlusal dysesthesia and perceptual distortion of the oral cavity.
5.1.7.1 Sensory Feedback of Dental Implants
The periodontal mechanoreceptors play a key role in sensing the force loaded on teeth (Trulsson 2006). In patients who have natural teeth replaced by dental implants, the periodontal mechanoreceptors are mostly removed during extraction. Therefore, the sensory feedback during occlusion may be altered. In an earlier study, Grigoriadis et al. (2011) investigated 13 patients with implant‐supported bridges, who had used them for at least one year, for their performance of chewing hard and soft food. They found that the patients with dental implants and the subjects with natural teeth did not significantly differ in the number of chewing cycles. The dentate subjects, but not the patients, showed a significant change in the amplitude and velocity of jaw movement when chewing hard food compared to soft food. The change in jaw movements suggested a better adaptation for food hardness in dentate subjects, but not patients with dental implants. Consistently, when chewing hard food, the patients showed a weaker increase in EMG activity, suggesting worse adaptation of the muscle activity to food hardness during mastication compared to dentated subjects (Grigoriadis et al. 2011).
Nevertheless, the loss of periodontal mechanoreceptors may not completely silence any sensory information from the implant. Sensory feedback of dental implants may be associated with ‘osseoperception’, which generally refers to as ‘a conscious perception of external stimuli transmitted via a bone‐anchored prosthesis by activation of neural endings and/or receptors in the peri‐implant environment’ (Abarca et al. 2006). Therefore, osseoperception is considered as the assembly of sensory feedback derived from mechanoreceptors in muscle, joint, mucosal, cutaneous, and periosteal tissues (Klineberg et al. 2005). So far, neuroimaging findings regarding osseoperception are still lacking. Habre‐Hallage et al. (2012) compared the brain activation of tactile stimulation on teeth in patients with implants and subjects with natural teeth. They found that stimulating implants activated an extensive bilateral cortical network outside the somatosensory areas, including the parietal, frontal and insular lobes (Habre‐Hallage et al. 2012). A recent functional near‐infrared spectroscopy (fNIRS) study also revealed activation of the S1 when subjects received vibratory tactile stimuli on both natural teeth and dental implants (Sekido et al. 2020). Due to the lack of psychophysical (e.g. the intensity of loading force) or functional (e.g. chewing performance) data, it is difficult to clarify the precise brain mechanisms of osseoperception.
5.1.7.2 Occlusal Dysesthesia
Occlusal dysesthesia (OD) refers to ‘a condition in which tooth contacts that are not clinically identifiable as premature contacts nor associated with other disorders’ but perceived as ‘disturbing or unpleasant’ for more than six months (Imhoff et al. 2020). For diagnosis of OD, symptoms derived from the disorders of the periodontal tissues, the dental pulp, the masticatory muscles or the temporomandibular joint need to be excluded (Imhoff et al. 2020). The neural mechanisms of OD have not been elucidated. Using fNIRS, Narita et al. (2019) found that both OD patients and healthy controls showed increased PFC activity during chewing compared to rest. However, OD patients showed a lower PFC activity during chewing compared to healthy controls (Narita et al. 2019). Notably, OD may present comorbidity with psychiatric disorders (Shinohara et al. 2020), and sleep restriction would influence the affective experience of occlusal sensation (Nishimori et al. 2019). Therefore, OD may not simply reflect a change in somatosensory processing. The brain mechanisms of cognitive–affective processing would play a key role in the development of OD.
5.1.7.3 Orofacial Perceptual Distortion
Perpetual distortion is a multisensory phenomenon commonly reported by patients as the feeling of ‘swollen’ on their painful face area (Dagsdóttir et al. 2018). In a pioneering study of this topic, Kothari et al. (2020) used repetitive TMS to manipulate the experience of perceptual distortion, as experimentally induced by local anaesthesia, on 52 healthy adults. Local anaesthesia was performed on the midface region innervated by the infraorbital nerve, and subjects were asked to report the perceived change in the size of the swollen area compared to the unaffected side. Based on single‐blinded randomization, half of the subjects received inhibitory rTMS at the somatotopic area of the S1 that represents their right face/lip after local anaesthesia. The researchers found a decreased magnitude in perceptual distortion immediately and 20 minutes after inhibitory rTMS compared to sham rTMS. Moreover, the effect was not induced by stimulation at the peripheral muscle or the cortical region other than the face/lip area (Kothari et al. 2020). The findings strengthen the link between cortical processing and individual experience of body image by highlighting the specificity between the S1 orofacial area and the location of experienced distortion.
5.1.8 Summary
- According to the model of body representation, the processing of oral somatosensation follows a hierarchical organization: the processing of somatosensation is associated with the transduction of physical stimuli via peripheral sensory receptors. Somatoperception is associated with the perception of an intraoral object via somatosensory information.
- Somaesthesis is a highly integrative and multisensory experience that consists of not only touch, chemical and gustatory information but also the appetitive status and affective feeling of the mouth.
- Oral somatosensation, as induced by tactile stimuli, is generally associated with an increased brain activation at the S1. Oral stereognosis, as an integrative experience related to oral somatoperception, may be associated with the brain mechanisms of both sensory and attentional–cognitive processing.
- Neuroimaging methods are adopted for investigating three clinical topics that are associated with the processing of oral somatosensation, i.e. osseoperception, occlusal dysesthesia and perceptual distortion of the oral cavity.
5.2 Brain Mechanisms of Gustation
5.2.1 Introduction
Gustation is a sensory modality derived from the taste buds in oral and pharyngeal epithelia when the receptors in taste buds are elicited by chemicals from food (Breslin 2013; Simon et al. 2006). Gustatory function is vital to survival because it influences our selection of foods and the physiological and metabolic processing of nutrients (Breslin 2013). Gustatory function has been less attended by dentists compared to other oral functions related to feeding behaviour (e.g. mastication and swallowing). However, there exists a noticeable relationship between gustatory function and the stomatognathic system. On the one hand, mastication plays a key role in maintaining normal gustatory function. Gustation is the chemical sense of the tastants deriving from food. The tastants are chemicals that activate taste receptor cells. The tastants need to be first released from food via mastication and then dissolved in saliva. Therefore, mastication relates to how foods are processed and tasted (Batisse et al. 2017). On the other hand, gustatory function and oral health are closely related. For example, both dysgeusia (i.e. an altered sense of taste) and burning pain are common symptoms of the burning mouth syndrome (BMS) (Imamura et al. 2019), and increased age is associated with increased thresholds of somatosensation and gustation (Heft and Robinson 2010). Therefore, gustatory function should be a clinical topic that deserves more attention from dental professionals. In this section, we outline the brain mechanisms associated with gustation. Especially, we highlight the role of affective–motivational processing of food, an issue highly relevant to gustatory and oral functions.
5.2.2 Brain Mechanisms of Gustation
Compared to other oral functions, such as mastication and swallowing, gustatory function has been relatively less explored by dentists. Nevertheless, this topic has been systematically studied from the early days of neuroscience and still a thriving field of research. In the following sections, we outline the current findings, primarily from animal research and human neuroimaging research, of the brain mechanisms of gustation.
5.2.2.1 Previous Findings from Animal Research
Animal models, from rodents to Drosophila, have provided valuable information about the neural mechanisms of the gustatory system, especially the neural circuitries of sensory transduction of taste stimuli. It has been clarified that taste percepts (i.e. a unique qualitative experience about what is perceived) are associated with the taste receptors encoded by specific genes. For example, the bitter taste is associated with the receptor genes T2Rs, and the sweet taste is associated with the genes T1R2/T1R3 (Breslin 2013; Simon et al. 2006). The signals from taste buds are transmitted to the nucleus tract solitarius (NTS) of the brainstem via the facial nerve, the glossopharyngeal nerve and the vagus nerve. The signals are subsequently relayed via two pathways: the ventral pathway to the amygdala and hypothalamus for autonomic and affective processing, and the dorsal pathway to the thalamus for the processing of taste qualities (Breslin 2013, Simon et al. 2006). Animal research has helped to clarify some ‘myths’ about taste. For example, in the tongue, there is no clear‐cut boundary between the areas responding to a specific taste stimulus. The tongue area sensitive to each taste stimulus is not spatially segregated (Scott 2005). Evidence from animal research also confirmed new percepts of taste. For example, taste receptor cells are found associated with the taste of fat, which may signify the richness of energy (Gilbertson 1998), and the taste of umami, which is induced by amino acids (Zhao et al. 2003). Finally, researchers have identified the insular cortex as the primary gustatory cortex (Maffei et al. 2012). Recent findings reveal that in the gustatory cortex, some neurons respond only to taste stimuli (i.e. a ‘unimodal’ neuron), and there exist the ‘bimodal neurons’ that respond to both tastants and odorants dissolved in water (Samuelsen and Fontanini 2017). The findings from animal research elucidate the multisensory processing of gustatory and olfactory information.
5.2.2.2 Meta‐analytical Findings from Human Neuroimaging Studies
While animal research contributes to elucidate the neural circuitries and molecular mechanisms of gustation, neuroimaging research focuses on human subjects and provides additional information about how the experience of taste is shaped. Notably, such an experience is not only associated with the transduction of taste stimuli but also with the affective–motivational processing of food. In a recent imaging meta‐analysis, Yeung et al. (2018) analyzed the findings from 34 neuroimaging studies that focus on the affective value, intensity and quality of receiving liquid/food stimuli of healthy subjects. When the subjects focused on rating the affective value of the taste stimuli, consistent activation was identified primarily in the bilateral anterior and middle insula, the precentral gyrus where the motor cortex resides and the thalamus. When it comes to the rating of intensity and quality of taste stimuli, consistent activation was identified in the anterior‐mid insula and mid‐dorsal insula, respectively (Yeung et al. 2018) (Figure 5.3). The findings suggest a key role of the insular cortex in gustatory processing from the sensory (i.e. rating of intensity and quality) to the affective–motivational (i.e. valence) level. Consistently, the findings from an earlier meta‐analysis also showed consistent activation at the insula when subjects perceived pure taste stimuli (Veldhuizen et al. 2011). The neuroimaging findings also confirm the conclusion from animal research, which identified the insular cortex as the location of the gustatory cortex (Maffei et al. 2012). In addition, neuroimaging studies have provided additional clues about gustatory processing by revealing a different pattern of activation in the insular subdivisions (Yeung et al. 2018) (Figure 5.3). The meta‐analysis revealed that the affective value of taste was associated with a consistent pattern of activation in the middle and anterior insula. In contrast, the quality of taste was primarily associated with activation in the mid‐dorsal insula (Yeung et al. 2018). The distinct pattern of activation corresponds to the functional and structural connectivity of the insula. The anterior and middle insula has a stronger connection with the PFC and the orbitofrontal cortex, primarily for cognitive–affective processing. In contrast, the posterior and middle insula has a stronger connection with the area for sensory processing (Wiech et al. 2014). Furthermore, the insula does not merely receive the gustatory information in bottom‐up processing. A recent fMRI study revealed that taste intensity was associated with top‐down (i.e., from the insula to the thalamus) information processing (Yeung et al. 2016). Together, the findings highlight multidimensional processing, including sensory, cognitive, affective and motivational aspects, of gustation.

Figure 5.3 Brain activation associated with gustation at the insula. A consistent pattern of brain activation is identified in the insular cortex for studies focusing on the quality, intensity and affective value of taste stimuli, respectively.
Source: Yeung et al. (2018). Reproduced with permission of Elsevier.
5.2.3 Recent Neuroimaging Findings of Gustation
Recent neuroimaging research has been focusing on the brain mechanisms of the qualitative experience of taste (e.g. sweet or bitter). As shown in Table 5.2, in the studies, specific tastants were used to induce distinct qualities of taste. In our daily life, the taste is perceived from consuming real food rather than the experimental tastants. The research scope has been extended to the relationship between gustation and food processing.
5.2.3.1 Brain Mechanisms Associated with Taste Stimuli
Recent neuroimaging findings support the notion that brain features may discriminate the different taste stimuli (Table 5.2). For example, the brain activation at the insula (Chikazoe et al. 2019) and the EEG activity of the delta‐frequency range (Wallroth et al. 2018) would be associated with the identification of taste quality. Using ultra‐high field fMRI, Chikazoe et al. (2019) found activation in the insula and frontal and parietal operculum discriminated between four basic qualities of taste (i.e. sweet, salty, bitter and sour), confirming the role of the insula in gustation. Compared to other taste stimuli, bitter and umami may be associated with activation at the primary gustatory cortex and subcortical regions (Meyer‐Gerspach et al. 2016). Compared to bitter, sweet may be associated with activation at the hippocampus, somatosensory cortex and orbitofrontal cortex (Sadler et al. 2020). Notably, the brain mechanisms may vary depending on individual dispositions of gustation. For example, when receiving umami stimuli, subjects with higher ability of identification of umami would show increased activation at the primary gustatory cortex compared to those with a lower ability (Han et al. 2018). fNIRS findings revealed that the addition of soy sauce and monosodium glutamate (MSG), which induces umami taste, is associated with brain activity at the frontal operculum (Onuma et al. 2018). Moreover, the addition of glutathione enhanced the taste intensity of the MSG. The effect of glutathione was associated with activation at the left ventral insula (Goto et al. 2016). Therefore, functional brain features may reflect the change of composition of taste stimuli and highlight the role of gustation in discriminating and selecting food nutrients.
5.2.3.2 Brain Mechanisms Associated with the Affective–Motivational Experience of Taste
It is noteworthy that in the neuroimaging studies of taste stimuli, brain activation was found in an extended area beyond the insula and the somatosensory areas. The brain mechanisms may reflect the affective–motivational processing of gustation. For example, when consuming food, individuals may feel an emotional experience, such as feeling the food tasty or bland. The emotional experience is associated with the motivation to pursue or avoid food. In a neuroimaging meta‐analysis, Brown et al. (2011) analyzed neuroimaging studies focusing on aesthetic appraisals of various sensory modalities, including vision, audition, olfaction and gustation. The brain activation when subjects appraised each of the sensory modalities was synthesized. They found that when appraising the taste stimuli to be pleasant or attractive, consistent brain activation was found not only in the anterior insula (which has been consistently identified for processing of taste quality) but also the anterior cingulate cortex and the lateral/medial orbitofrontal cortex (Brown et al. 2011). Notably, the authors found a common activation of the anterior insula across different sensory modalities, suggesting its role in the appraisal of the affective valence of sensory experience (Brown et al. 2011).
Table 5.2 Neuroimaging research on brain mechanisms of taste or food stimuli (since 2015).
Source | Participants | Methods |
---|---|---|
Eldeghaidy et al. (2021) | 12 thermal tasters and 12 thermal non‐tasters | fMRI/sweet stimuli delivery at cold vs. ambient temperatures |
Sadler et al. (2020) | 85 healthy adults | fMRI/a probabilistic selection task of sweet and bitter stimuli |
Chikazoe et al. (2019) | 31 healthy adults | fMRI/sweet, salty, bitter and sour stimuli |
Kono et al. (2018) | 18 healthy adults | EEG/five basic taste stimuli and capsaicin of different concentrations |
Han et al. (2018) | 15/15 subjects with high/low umami identification ability | fMRI/umami and salty stimuli |
Onuma et al. (2018) | 44 healthy adults | fNIRS/salty stimuli w/wo umami stimuli or the odour of soy sauce |
Wallroth et al. (2018) | 16 healthy adults | EEG/salty, sweet, sour or bitter stimuli |
Goto et al. (2016) | 26 healthy adults | fMRI/umami and salty stimuli w/wo glutathione |
Meyer‐Gerspach et al. (2016) | 12 healthy adults | fMRI/bitter, sweet, sour, salty and umami stimuli; working memory processing |
Hort et al. (2016) | 12 thermal tasters and 12 thermal non‐tasters | fMRI/sweet stimuli with no CO2 and low and high CO2 levels |
Mascioli et al. (2015) | 11 healthy adults | fMRI/taste and tactile stimulation on the unilateral side of the tongue |
Frank‐Podlech et al. (2019) | 11 normal‐weight adults | rs‐fMRI/before and after having high‐ vs. low‐fat yogurt |
Smeets and de Graaf (2019) | 21 regular beer drinkers | fMRI/anticipating and consuming beer, non‐alcoholic beer and carbonated water |
Wistehube et al. (2018) | 25 patients with chronic brain lesions (25 healthy controls) | fMRI/milkshakes with different sugar or fat content |
Eiler II et al. (2018) | 74 adults with a positive or negative family history of alcoholism | fMRI/sweet stimuli of different sucrose concentrations |
Shearrer et al. (2018) | 53/55 adolescents who were at high/low risk of obesity | fMRI/milkshakes with different sugar or fat content |
Dalenberg et al. (2017) | 45 healthy adults | fMRI/familiar drinking products or unfamiliar oral nutritional supplements |
Eldeghaidy et al. (2016) | 17 healthy adults | fMRI/flavoured no‐fat control stimulus or flavoured fat stimulus after the prior consumption of high‐fat meal or noncaloric water load |
van Rijn et al. (2015) | 30 healthy adults | fMRI/three food stimuli (sweet/no energy, non‐sweet/energy and sweet/energy) in hunger and satiety status |
Ebisch et al. (2015) | 25 healthy adults of high or low emotion susceptibility |
fMRI/flavour stimuli with affective valences: neutral (water), pleasant (apple nectar) or unpleasant (quinine) |
Mazzola et al. (2017) | 221 patients with epilepsy | Stimulation of the insular cortex with implanted depth electrodes |
Notes: EEG: electroencephalography; fMRI: functional magnetic resonance imaging; fNIRS: functional near‐infrared spectroscopy; rs‐fMRI: resting‐state functional magnetic resonance imaging.
5.2.4 Neuroimaging Findings of Food Perception
There has been an increasing number of neuroimaging studies on food perception, which is associated with the processing of oral somatosensory and gustatory information. Moreover, food perception can be modified by oral functions (e.g. mastication) (Batisse et al. 2017). Recent neuroimaging studies disclosed that the brain mechanisms of processing food may be associated with the components of food rather than its taste. By investigating the resting‐state functional connectivity (rsFC) before and after a high‐ or low‐fat diet, researchers found that subjects with a higher sensitivity to fat, as assessed by a standardized oral sensitivity test, showed a stronger rsFC between homeostatic regions (e.g. the hypothalamus) and limbic areas (Frank‐Podlech et al. 2019). Another study investigated the difference of perception and brain activation between drinking alcoholic beer and non‐alcoholic beer in regular beer drinkers. Their ratings of liking and desire to drink did not significantly differ between these two types of beers. However, their brain activation after swallowing revealed increased activation related to the taste of alcoholic beer at the PFC and the anterior insula compared to the taste of non‐alcoholic beer (Smeets and De Graaf 2019). When investigating the perception of sugar and fat, researchers found that patients with brain damage showed an impaired fat perception but not sugar perception. Moreover, the impaired fat perception was associated with lesions of the anterior insula and frontal operculum (Wistehube et al. 2018). Notably, recent neuroimaging findings highlight the importance of individual factors in the perception of food stimuli. When taking the solution of various concentrations of sucrose, subjects with a family history of alcoholism showed a greater activation at the bilateral amygdala when the sucrose concentration was low (Eiler II et al. 2018). Another fMRI study revealed that adolescents with a high risk of obesity (based on parental obesity status) showed increased activation in caudate, gustatory and oral somatosensory areas when taking the high‐sugar milkshake compared to the tasteless solution (Shearrer et al. 2018). In general, the findings confirm that the insula plays a key role in gustatory perception (Shearrer et al. 2018; Smeets and De Graaf 2019; Wistehube et al. 2018) and highlight the role of other regions in shaping one’s perception of food components, including the PFC and the amygdala, which both show a substantial functional and structural connection with the anterior insula (Wiech et al. 2014).
Several studies focus on the association between affective–motivational factors and food perception (Table 5.2). When subjects took the drinking products that they were familiar with, the rating of pleasantness was associated with the brain network consisting of the amygdala, ventral prefrontal, insular, striatal and parahippocampal area (Dalenberg et al. 2017). Upon subjects finished consuming a high‐fat meal, decreased activation in the amygdala was identified when more fat stimuli were delivered. The findings suggest the brain mechanism for an effect of ‘satiety’ after a meal (Eldeghaidy et al. 2016). Here, the change of insular activation may not solely reflect a change in taste processing but also a change in the motivation for acquiring food. In addition to fat, our sense of energy intake (e.g. the sugar content of food) is associated with a hunger state. The interaction between hunger vs. satiety status and perception of the energy content of foods may be associated with the activation of the anterior insula and the thalamus (Van Rijn et al. 2015). Finally, subjects with low emotional susceptibility revealed increased activation in the anterior insula to pleasant and unpleasant flavour stimuli (e.g. apple nectar and quinine) compared to neutral stimuli (Ebisch et al. 2015). Together, the neuroimaging findings suggest that the brain mechanisms of food perception are associated with widespread brain regions of affective–motivational processing, which remained not fully elucidated.
5.2.5 Summary
- Gustation is a sensory modality derived from the taste buds in oral and pharyngeal epithelia when the receptors in taste buds are elicited by chemicals from food. Animal models, from rodents to Drosophila, have provided valuable information about the neural mechanisms of the gustatory system, especially about the neural circuitry of sensory transduction of taste stimuli.
- Neuroimaging findings suggest a key role of the insular cortex in gustatory processing from the sensory (i.e. rating of intensity and quality) to the affective–cognitive (i.e. valence) level.
- Neuroimaging evidence reveals the pattern of brain activation specific to a distinct quality of taste. The brain mechanisms may reflect the processing of gustation as associated with the emotional and motivational experiences.
- There has been an increasing number of neuroimaging studies on food perception, which is associated with the processing of oral somatosensory and gustatory information. Recent neuroimaging findings suggest that food perception may be associated with the insula, the PFC and the amygdala, which both show a substantial functional and structural connection with the insula.
5.3 Cognitive–Affective Issues of Oral Sensory Functions
5.3.1 Introduction
A common analogue for a dentist’s job is the work of a watchmaker. To the general public, both are professionals who master fix complex structures. Such an analogue is partly wrong because dental patients, unlike watches, will actively engage with the dentists during treatment. Therefore, dentists always need to pay attention to the emotional and behavioural responses of patients, in addition to their teeth. These emotional and behavioural responses are shaped by a complex set of mental functions, including perception, attention, motivation and emotion. Following somatosensation and gustation, this section focuses on how these cognitive–affective functions shape our experience of oral conditions. Specifically, we outline the current understanding of perception, attention, motivation and emotion from the perspective of cognitive neuroscience and highlight the association between oral sensorimotor functions and these cognitive–affective functions.
5.3.2 Perception
As the saying goes, seeing is believing. We know the world by a variety of sensations. In terms of cognitive neuroscience, sensation refers to the activation of peripheral receptors and the translation of environmental information into neural signals (Gazzaniga et al. 2019). Perception, in contrast, refers to the process that one constructs the mental representation of the original process (Gazzaniga et al. 2019). Finally, the mental representation needs to be interpreted by integrating information from other mental functions, such as memory and emotion. In general, our experience is shaped by such a flow of information processing. However, such a ‘flow’ of information does not mean a one‐direction pathway with a fixed neural circuitry. As discussed in the following sections, there exist complicated mechanisms for perceptual processing.
5.3.2.1 Bottom‐up and Top‐down Processing of Perception
From an evolutionary perspective, we focus on the information that is useful for increasing the chance to survive. Therefore, there are two major roles in perceptual processing. Firstly, it constructs veridical information about the environment. Secondly, what is perceived should update our expectation about the environment (Press et al. 2020b). By doing so, individuals can respond effectively to the environment and adapt efficiently to changes in the environment. Our mental functions help us to construct a world in our mind based on the information that we sense from the environment. However, there can be a gap between sensing and perceiving. For example, when dental patients notice the presence of a ‘forceps‐like instrument’, they may focus on the sharp edge of the instrument and associate it with some invasive procedure that they have experienced. In other words, the perception of ‘what the instrument is like’ is associated with not just sensory information but also one’s expectation, memory and knowledge. In terms of cognitive neuroscience, perception is associated with both top‐down and bottom‐up processing. The former highlights the neural processing of intrinsic (personal) factors, such as goal planning and selective attention (Engel et al. 2001). The latter highlights the neural processing of extrinsic (environmental) factors, such as the physical features of stimuli (Gazzaniga et al. 2019) (Figure 5.4a). It is noteworthy that the concept of top‐down and bottom‐up processing was originally proposed in the literature of visual perception to explain how visual information is organized (Rauss and Pourtois 2013). For example, the bottom‐up processing focuses on detecting the local features of a target stimulus and its difference from neighbouring stimuli. The top‐down processing focuses on our inner goal, which directs how we perceive the stimuli. The difference between top‐down and bottom‐up processing does not imply the directionality of neural pathways. Both ascending pathways (from peripheral to central nervous system) and descending pathways would jointly participate in the processing (Rauss and Pourtois 2013).

Figure 5.4 Basic concepts of perceptual processing. (a) Top‐down processing highlights the neural processing of intrinsic (personal) factors, such as one’s goal planning, on the formation of perceptual experience. The bottom‐up processing highlights the neural processing of extrinsic (environmental) factors, such as the physical features of stimuli, on the formation of perceptual experience. (b) In predictive coding, the sensory inputs that we receive from the real world are compared with our prediction of the sensory outcomes. A mismatch (i.e. ‘prediction error’) occurs when our prediction does not fit the outcome we perceive. The prediction error is associated with attentional bias and learning. For example, we may pay more attention to an unexpected event compared to an expected one.
5.3.2.2 Predictive Coding of Perception
The concept of top‐down and bottom‐up processing implies a hierarchical organization of perceptual processing, consisting of a ‘higher‐level’ processing of integrating information (e.g. to expect what to find) and a ‘lower‐level’ processing of detailed stimulus information (e.g. to find the features of an object) (Rauss and Pourtois 2013) (Figure 5.4a). In recent years, researchers have formulated theories for describing the interaction between these two conceptual levels. Cumulating evidence suggests that the match (or mismatch) between the higher‐ and the lower‐level processing plays a dominant role in perception (Figure 5.4b). The nervous system is critical for matching the prediction generated internally to external stimulation (Rauss and Pourtois 2013). Our brain is not merely an organ that passively receives stimuli from the world. It is a calculator or an active ‘inference machine’ (Friston 2010), which anticipates sensory inputs and forms predictions about the incoming events (Barrett and Simmons 2015). When we are sensing the world, we pay more attention to the presence of the events that we expect to find compared to those we do not predict to find (Box 5.1). Sensory inputs that we receive from the real world are compared with the prediction. If a mismatch (i.e. ‘prediction error’) is detected (i.e. something ‘out of expectation’ occurs), our attention may be biased to the unexpected sensory inputs (Figure 5.4b). When something unexpected is detected, the old prediction needs to be updated so that new information can be integrated (Press et al. 2020b). In general, such perceptual processing can be deemed as a process of learning driven by prediction error (Corlett 2020; Press et al. 2020a, b).

VIDEdental - Online dental courses
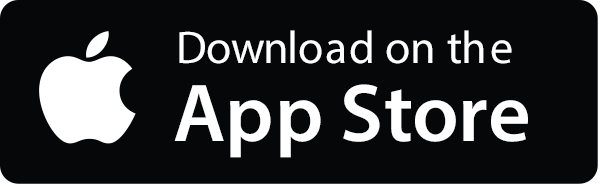
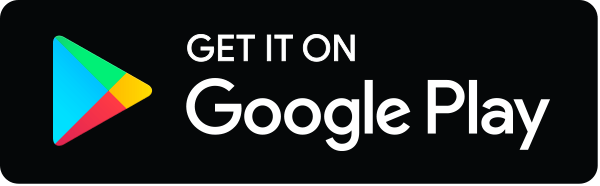