6
Brain Mechanisms of Pain and Anxiety of Dental Patients
6.1 Brain Mechanisms Related to Pain
6.1.1 Introduction
The definition of pain has been recently updated by the International Association for the Study of Pain (IASP) as ‘an unpleasant sensory and emotional experience associated with, or resembling that associated with, actual or potential tissue damage’ (IASP 1994, 2020). The definition highlights that pain, as a subjective experience of multiple dimensions (e.g. sensory–discriminative, affective–motivational and cognitive dimensions), differs from nociception, which refers to ‘the neural process of encoding noxious stimuli’ (IASP 1994, 2020). In line with the terminology of pain, modern neuroimaging research on pain focuses on not only the processing of nociception but also the attentional, cognitive and emotional processing of pain (Apkarian et al. 2009; Davis et al. 2017; Mouraux and Iannetti 2018). In the following sections, we first outline the theoretical framework of brain mechanisms of pain, which has been substantially influenced by the concept of ‘neuromatrix’ (Melzack 1990). Secondly, we summarize the brain mechanisms of acute pain based on neuroimaging research. Thirdly, the brain mechanisms of chronic pain, especially the difference of brain activation between chronic and acute pain, are outlined. Finally, we discuss a hotly debated question in translational medicine, i.e. if one can find an objective neuroimaging marker to predict one’s feeling about pain.
6.1.2 From the Neuromatrix of Pain to ‘Pain Matrix’
From a historical perspective, the neuromatrix theory of pain was conceptualized even earlier than the trend of neuroimaging research on pain. Originally, Melzack (1990) proposed a neural network that subserves body sensation. He termed the network the ‘body‐self‐neuromatrix,’ which ‘integrates multiple inputs to produce the output pattern that evokes pain’ (Melzack 1999). Neurologically, this body‐self‐neuromatrix ‘comprises a widely distributed neural network that includes somatosensory, limbic and thalamocortical components’ (Melzack 1999). The proposal highlights that the brain mechanisms of pain are not just about the processing of sensory–discriminative but also the affective–motivational and evaluative–cognitive information of pain (Melzack 1990, 1999, 2001). The neuromatrix theory of pain extends previous research findings of ‘nociceptive pathways,’ which focus on the transmission of noxious stimuli (Giesler Jr. et al. 1994). The neuromatrix theory highlights a dynamic role of our brain in shaping pain experience rather than a static receiver of the nociceptive signals from the body.
While the framework of pain neuromatrix highlights the multi‐dimensionality of pain, it is not a ‘theory’ with enough details for explaining all the mental processes about pain (Derbyshire 2000). For example, the neuromatrix is conceived to function as a network, but what are the components included in the network? How does each component connect with the other? These are all the questions that remained unanswered. The framework was later evolved into the concept of pain matrix, which can be loosely defined as ‘a group of brain structures jointly activated by painful stimuli’ (Garcia‐Larrea and Peyron 2013). The definition provides an operational basis for identifying the pain matrix (i.e. by identifying the loci of brain activation). Still, the association between these loci and pain, especially its causal mechanisms, has remained unclear. Furthermore, though many neuroimaging studies adopted the concept of the pain matrix, there exists a great variation of its definition across studies. For example, different neuroimaging studies may identify the ‘pain matrix’ by finding brain activation at different regions (Davis et al. 2017; Derbyshire 2000; Mouraux and Iannetti 2018). In addition to the research purpose, there is also a practical need for elucidating the association between pain and the brain. Clinically, the symptoms and signs of chronic pain differ from those of acute pain, and the pain derived from neuropathic mechanisms (e.g. trigeminal neuralgia [TN]) would differ from that derived from nociceptive mechanisms (e.g. a toothache from pulpitis). The patients with chronic pain show an altered affective–motivational experience compared to the patients with acute pain (Malfliet et al. 2017). Therefore, it is not surprising that the brain mechanisms of acute pain would be different from those of chronic pain, which may be more associated with affective–cognitive processing of pain; and the brain mechanisms associated with neuropathic pain may be associated with the mechanisms of neural injury and repair. Therefore, it would be questionable if the ‘pain matrix’ identified by experimental pain can be generalized to elucidate the brain mechanisms of chronic pain. In the following sections, we respectively investigate the brain mechanisms of acute and chronic pain.
6.1.3 Brain Mechanisms Related to Acute Pain
The recent advancement of neuroimaging has offered an excellent opportunity to directly observe the brain activation associated with pain via a non‐invasive approach in human subjects. Following the framework of pain matrix, researchers can map the brain network by investigating the loci of brain activation when subjects received painful stimuli. In an earlier review, Derbyshire reported that the anterior cingulate cortex (ACC), the primary and secondary somatosensory cortices, the insula and the thalamus showed consistent activation across a variety of pain research of tonic or phasic pain (Derbyshire 2000). Subsequently, several meta‐analyses have been published by pooling the patterns of brain activation derived from the research on experimental pain (Burns et al. 2016; Duerden and Albanese 2013; Farrell et al. 2005; Jensen et al. 2016; Peyron et al. 2000; Tanasescu et al. 2016) (Figure 6.1a). In general, the studies identified that during the stimulation by experimental pain, the primary somatosensory cortex (S1) and secondary somatosensory cortex (S2), the insula, the cingulate cortex and the thalamus have been consistently identified for their activation (Figure 6.1a). The findings are consistent with the results of animal research, which has identified the role of the thalamocortical circuitry in pain processing. Specifically, the lateral pathway of the circuitry participates in the processing of sensory–discriminative information of pain, including the S1 and the S2. The medial pathway, in contrast, participates in the processing of cognitive–affective information of pain, including the insula and the cingulate cortex pain (Groh et al. 2018). The results reveal that the processing of acute pain is more likely to engage with a ‘network’ of multiple brain regions. However, it does not mean that these brain regions are only for pain processing. For example, the thalamus relays sensory information from both nociceptive and non‐nociceptive stimuli, and the anterior insula and the ACC are associated with multiple brain functions, from attentional control to processing of interoception (Simmons et al. 2013). Notably, there is a great variation in the pattern of regional activation across the studies. For example, activation in the limbic system, such as the hippocampus and the amygdala, was not consistently identified across all the studies. The inconsistent pattern of activation of these regions may reflect the fact that people are much different in their cognitive–affective experience of pain (e.g. different feelings of threat towards pain).

Figure 6.1 Brain activation associated with pain processing. (a) The brain regions commonly reported in functional magnetic resonance imaging (fMRI) studies of noxious stimuli. The figure only displays the relative position and size of the brain regions, not depicting the anatomical details. Note that the insular cortex (bounded by a dashed line) is covered by the frontal, parietal and temporal operculum. (b) Meta‐analytical findings of the brain activation of experimental orofacial pain in healthy subjects. Increased activation is consistently found in the posterior mid‐cingulate cortex (pMCC), the PPC, the insula, the thalamus, the S1 and the S2. Decreased activation is consistently found in the primary motor cortex (M1) and the S1.
Source: Ayoub et al. (2018). Reproduced with permission of Elsevier.
The same findings have been replicated in recent neuroimaging meta‐analyses about experimental orofacial (Ayoub et al. 2018; Lin et al. 2014b). For example, Lin et al. (2014b) have analyzed the pattern of the brain activation of six studies of electrical stimulation on the teeth of healthy subjects. They found that activation at the thalamus, the somatosensory cortex, the insula, the cingulate cortex and the dorsolateral prefrontal cortex (PFC) were consistent in the studies. The pattern of activation generally corresponds to the medial and lateral nociceptive systems (Groh et al. 2018). When healthy adults received acute toothache elicited by electrical stimuli, the activation of the anterior insula and the ACC reflected the intensity of pain (Brügger et al. 2012). Furthermore, the brain activation of the posterior insula reduced when dental pain was relieved by a mental nerve block (Meier et al. 2015). Notably, the presence of the dorsolateral PFC may signify the importance of attentional and cognitive processing during painful stimulation (Lin et al. 2014b). Another meta‐analysis by Ayoub et al. (2018) also confirmed the role of similar brain regions when they investigated orofacial pain induced by electrical, mechanical, thermal or chemical stimuli. In the study, the PFC was not reported as a consistently activated region, perhaps due to the variations in experimental conditions. In general, the findings revealed that brain regions for sensory, cognitive and emotional processing together take part in the processing of pain, highlighting the multi‐dimensionality of pain (Figure 6.1b).
6.1.4 Cognitive–Affective Factors Related to Acute Pain
It is widely known that cognitive–affective factors, such as the expectation related to pain and attention of pain, may modulate our pain (Wiech 2016). For example, Ploghaus et al. (2001) found that when subjects received noxious stimuli of the same intensity, they perceived greater pain when the stimuli were associated with higher anxiety compared to the stimuli associated with lower anxiety. Furthermore, the anxiety‐potentiating effect on pain is associated with brain activation at the hippocampus (Ploghaus et al. 2001). Likewise, Wiech et al. (2010) found that when subjects received noxious stimuli calibrated at the detection threshold of pain, they were more frequently to rate the stimuli as ‘painful’ in the conditions when they felt that the stimuli were threatening compared to the conditions when they felt the stimuli were safe. Increased threat value and increased pain are both associated with brain activation at the anterior insula (Wiech et al. 2010). Tracey et al. (2002) reported that when subjects received painful thermal stimuli vs. non‐painful warm stimuli, they perceived the stimuli as less painful when they were not attending to pain compared to the condition when they were attending to pain. Notably, the periaqueductal grey (PAG) showed increased activation in the non‐attentive conditions compared to the attentive conditions (Tracey et al. 2002). In the functional magnetic resonance imaging (fMRI study by Ploner et al. (2011)), subjects received painful stimuli in accompany with the following conditions: (i) an ‘attention’ condition that they counted the number of stimuli they received and (ii) an ‘emotional’ condition that they watched pictures of different emotional valence. Subjects perceived greater pain when they attended to pain and watched pictures with negative emotional context. Both the attention and emotional effects were associated with an increased brain activation at the anterior insula, and the anterior insula showed a distinct connection to the brain networks of attention and emotional processing (Ploner et al. 2011). Together, the findings suggest that by manipulating the cognitive–affective factors, such as the threat value related to pain and attention of pain, experimenters can modulate the pain intensity perceived by subjects.
The brain mechanisms of cognitive and emotional processing of pain have been gradually clarified by recent neuroimaging meta‐analyses (Table 6.1). Some experience of ‘pain’ may arise even without direct noxious stimuli. For example, the pain derived from empathizing with other’s pain (e.g. watching another person being cut in fingers) is associated with activation at the anterior insula and the ACC (Lamm et al. 2011). The frontoparietal regions are associated with pain empathy of different body parts and the visuospatial perspective (e.g. first or third‐person) (Jauniaux et al. 2019). Research of event‐related potential (ERP) also revealed the association between the frontoparietal regions and pain empathy (Coll 2018). Notably, a recent meta‐analysis revealed a weaker association between placebo analgesia and the neurological signature of nociceptive pain, a neuroimaging marker for nociceptive pain processing (Wager et al. 2013). The findings suggest that the brain mechanisms of pain modulation by placebo may be largely independent of bottom‐up nociceptive processing (Zunhammer et al. 2018). Neuroimaging meta‐analysis also identified the brain mechanisms of pain modulation by hypnotic suggestions, including hypnotic analgesic, pleasant or depersonalization suggestions (HASs). When subjects received experimental pain, HASs were associated with increased activation at the ACC, the insula and PFC, as well as decreased activation in the thalamus compared to the control condition (Del Casale et al. 2015). Activation of the posterior insula and the parietal lobe can be identified when individuals perceived painful vs. non‐painful cold stimuli (King and Carnahan 2019). The insular activation is also found when subjects perceived itch (Roberts et al. 2019). Furthermore, the activation at the insula, the cingulate cortex and the frontoparietal regions is also identified when subjects anticipated (rather than received) pain (Palermo et al. 2015). These recent findings, again, highlight the role of cognitive–affective processing in the brain mechanisms of acute pain.
Table 6.1 Recent meta‐analytical findings on neuroimaging research on pain (since 2015).
Source | Studies | Methods |
---|---|---|
Jauniaux et al. (2019) | 86 fMRI studies of pain empathy of different visual cues, visuospatial and cognitive perspectives | ALE |
King and Carnahan (2019) | 28 fMRI/PET studies of innocuous and noxious cold exposure | ALE |
Roberts et al. (2019) | 11 fMRI experiments of experimentally induced itch | ALE |
Ayoub et al. (2019) | 21 fMRI/PET/ASL‐MRI studies of experimental pain and 28 studies of chronic pain | ALE |
Coll (2018) | 40 EEG studies of empathy of pain in others | Random effects model |
Zunhammer et al. (2018) | 20 fMRI studies of placebo effects | Random effects model |
Ayoub et al. (2018) | 30 MRI/PET functional and structural studies of experimental orofacial pain and chronic orofacial pain | ALE |
Tatu et al. (2018) | 55 VBM studies of chronic pain | ALE |
Chang et al. (2018) | 67 studies (TMS/MRI/EEG/MEG/MRS/ PET) of patients with chronic pain | Random effects model |
Yuan et al. (2017) | 10 VBM studies of chronic back pain | Seed‐based d mapping |
Jia and Yu (2017) | 8 sMRI and 5 fMRI studies of migraine | ALE |
Tanasescu et al. (2016) | 266 fMRI studies of experimental cutaneous pain | Modified ALE‐based algorithm |
Burns et al. (2016) | 25 fMRI/PET/TMS/EEG studies of acute muscle pain | Random effects model |
Jensen et al. (2016) | 138 fMRI/PET studies of pain in healthy individuals and 32 studies of pain in patients | ALE |
Dehghan et al. (2016) | 37 MRI/PET/SPECT/DTI/MRS/EEG/MEG studies of fibromyalgia syndrome | ALE |
Del Casale et al. (2015) | 8 functional neuroimaging studies of pain perception under hypnosis | ALE |
Palermo et al. (2015) | 19 fMRI studies of pain anticipation | ALE |
Dai et al. (2015) | 9 VBM studies of migraine | ES‐SDM |
Pan et al. (2015) | 10 VBM studies of neuropathic pain | ES‐SDM |
Notes: ALE: activation likelihood estimation; ASL: arterial spin labelling; DTI: diffusion tensor imaging; EEG: electroencephalography; ES‐SDM: effect size‐signed differential mapping; fMRI: functional magnetic resonance imaging; MEG: magnetoencephalography; MRI: magnetic resonance imaging; MRS: magnetic resonance spectroscopy; PET: positron emission tomography; sMRI: structural magnetic resonance imaging; SPECT: single photon emission computed tomography; TMS: transcranial magnetic stimulation; VBM: voxel‐based morphometry.
6.1.5 Brain Mechanisms Related to Chronic Pain
Unlike acute pain, chronic pain is usually stimulus‐independent and occurs spontaneously. Therefore, different approaches have been adopted to study the brain mechanisms of chronic pain. Firstly, if we want to know the brain activation associated with ongoing pain, we may ask patients to continuously rate how pain fluctuates during the imaging session (Baliki et al. 2006, 2010). Secondly, if we are interested in the structural features associated with pain, we would focus on the link between structural features and patients’ self‐reported ratings. Because pain is a subjective experience, self‐report is still the gold standard for assessing individual pain (Davis et al. 2017).
Previous studies have revealed that the patterns of brain activation vary between different types of clinical pain. For example, patients with chronic back pain (CBP), post‐herpetic neuralgia (PHN), complex regional pain syndrome (CRPS), osteoarthritis of the knee (OA) and pelvic pain (PP) showed different patterns of brain activation during imaging (Apkarian et al. 2009; Mansour et al. 2016). These chronic pain‐related patterns consistently showed a distinct activation at the PFC (Apkarian et al. 2009). Baliki et al. (2006) reported that in the patients with chronic low‐back pain, sustained high pain was associated with increased activation in the medial prefrontal cortex (mPFC), which are widely considered as the brain regions associated with affective–motivational processing. In general, patients with chronic pain showed altered brain features not just in the sensorimotor network but also in the brain network associated with affective and motivation processing (Baliki and Apkarian 2015; Mitsi and Zachariou 2016). Chronic pain is also associated with alterations in the functional connectivity of brain networks (Figure 6.2). Among these networks, the default mode network (DMN) has been widely investigated for chronic pain. The DMN mainly includes frontal and posterior midline (e.g. the ventral mPFC and the posterior cingulate cortex (PCC)) and the inferior parietal lobule (Buckner et al. 2008). During a resting state, higher functional connectivity was found between the brain regions of the DMN. Interestingly, the DMN connectivity reduces when subjects are actively engaged in an experimental task. In other words, the DMN shows stronger connectivity when individuals are not disturbed by external stimuli (i.e. during a resting state) compared to the condition when they are interacting with the environment (e.g. during a task‐based fMRI study). Baliki et al. (2008a) investigated the DMN in patients with CBP and found that when the patients were performing an attention task, they showed a reduced deactivation in the brain regions of the DMN. Furthermore, a recent neuroimaging meta‐analysis revealed that patients with chronic pain showed consistently less activation in the right anterior hippocampus, and in patients with CBP, there was a weaker resting‐state functional connectivity between the anterior hippocampus and the mPFC compared to healthy controls (Ayoub et al. 2019). The findings suggest that changes of the default (i.e. task‐free or stimulus‐independent) network of the brain may be associated with persistent and spontaneous pain in patients with chronic pain.

Figure 6.2 Functional networks associated with chronic pain.
Source: Davis et al. (2017) with permission of Springer Nature under the terms of the Creative Commons CC BY 4.0 License.
In addition to the fMRI studies, structural magnetic resonance imaging (sMRI) studies focus on identifying the association between brain features and self‐report findings or clinical symptoms. Meta‐analytic findings revealed that patients with chronic pain showed an increased grey matter volume (GMV) mainly at the bilateral thalamus and the bilateral basal ganglia, and a decreased GMV mainly at the bilateral ACC and dorsolateral PFC compared to healthy controls (Tatu et al., 2018). Patients with migraines showed a decreased GMV in the posterior insula, the PFC and the ACC (Dai et al. 2015; Jia and Yu 2017). Likewise, in patients with chronic low back pain, there is a decreased GMV in the medial frontal cortex and the ACC (Yuan et al. 2017). In neuropathic pain, patients showed decreased GMV in multiple regions, including the anterior insula and lateral frontal gyrus. There is also an increased GMV in the posterior insula and medial frontal gyrus (Pan et al. 2015). The participation of the primary motor cortex (M1) is less clear‐cut in patients with chronic pain (Chang et al. 2018). In patients with fibromyalgia syndrome, consistent changes are found not only in the somatosensory area but also in the insula and the amygdala (Dehghan et al. 2016). Moreover, the experience of chronic pain may be associated with the plastic effect on grey matter morphology. In a longitudinal MRI study, Seminowicz et al. (2011) investigated patients with chronic low back pain before and six months after receiving treatment. They found that before treatment, the patients showed a thinner cortical thickness at the dorsolateral PFC, the insula and the ACC, compared to the healthy controls. After treatment, the patients showed a regain in the thickness in the dorsolateral PFC, which was associated with a reduction of pain (Seminowicz et al. 2011). Furthermore, a recent meta‐analysis on sMRI studies of chronic pain revealed that among the brain regions showing alterations between patients and healthy controls, there exist co‐alterations between some pair of regions. Brain networks were established according to the spatial distribution of co‐altered regions, respectively, for the regions with increased and decreased GMV. Notably, the researchers also identified more regions with decreased GMV (in patients vs. healthy controls) than regions with increased GMV. The anterior insula, the ACC and the posterior insula play a key role in the co‐altered network of decreased GMV (Tatu et al. 2018). The findings suggest that structural alterations of the salience network may play a key role in chronic pain.
6.1.6 Cognitive–Affective Factors Related to Chronic Pain
Since chronic pain is associated with various cognitive and emotional experiences, it is not surprising that the pattern of brain activation associated with chronic pain will show a substantial overlap with the brain activation associated with emotional and motivation processing, which is not necessarily pain‐related (Davis et al. 2017). In the following sections, we briefly discuss two topics of cognitive–affective processing of pain, i.e. the brain mechanisms of pain modulation and reward processing of pain.
6.1.6.1 Modulation and Chronification of Pain
The pain we perceive does not fully reflect the intensity of nociceptive stimuli. In other words, the stimuli of the same nociceptive intensity may be perceived differently due to the modulation from the central nervous system (CNS), in which the brain plays a critical role. As we have seen in the preceding sections, nociceptive stimuli can be perceived as stronger or weaker pain in different experimental conditions, which vary between the threat value related to pain or attention of pain (Ploner et al. 2011; Tracey et al. 2002; Wiech et al. 2010). Neuroimaging findings have identified activation of the brainstem and the medulla, including the PAG, during pain modulation by cognitive–affective processing (Lin et al. 2014a; Tracey et al. 2002). The findings correspond to the descending pathway of pain modulation identified from animal research (Ossipov et al. 2010). Furthermore, the neuroimaging studies gradually revealed how the higher cortex, including the PFC, participates in pain modulation. Neuroimaging research helps to clarify the role of the PFC in pain modulation and its contribution to the chronification of pain. For example, an earlier longitudinal study of patients with sub‐acute back pain revealed that the white matter fractional anisotropy (FA) assessed at the baseline predicted the persistence of pain over the next year (Mansour et al. 2013). Moreover, in the subjects whose pain recovered after one year, the regional FA of the mPFC was positively correlated with the FA of the nucleus accumbens (NAc) as well as the functional connectivity between NAc and mPFC. Together, the findings highlight that the mPFC may play a key role in mediating antinociceptive effects, partly via the PAG (Ong et al. 2019), and contribute to the chronification of pain.
6.1.6.2 Chronic Pain and Reward Processing
The mesolimbic pathway, which consists of the NAc and the ventral tegmental area (VTA), is one of the major dopaminergic pathways that play a key role in reward and motivation processing (Serafini et al. 2020). Alterations in the mesolimbic pathway may contribute to reorganizing the neocortex into a chronic pain state (Baliki and Apkarian 2015). The activation of reward circuitry may play a key role in the experience of chronic pain (Mitsi and Zachariou 2016). The PFC may relate to the mesolimbic pathway and plays a key role in motivation and reward processing in chronic pain patients. For example, in patients with sub‐acute pain lasting for one year, decreased GMV at the NAc and increased functional and structural connectivity with the PFC have been noted (Baliki et al. 2012; Serafini et al. 2020). When receiving acute painful thermal stimuli, healthy subjects showed higher activation in the NAc compared to patients with CBP (Baliki et al. 2010). Notably, in the patients, the functional connectivity between the NAc and the mPFC was positively correlated with the intensity of their chronic pain (Baliki et al. 2010). Together, the findings highlight the key role of the interaction between pain modulation and reward processing in chronic pain. Patients with rheumatoid arthritis showed increased functional connectivity between the SMA, the mid‐cingulate cortex, the primary somatosensory area, the PFC and the insula (Flodin et al. 2016). Notably, in the patients with chronic pain, thinning of cortical thickness at the PFC was noted (Seminowicz et al. 2011). In general, the findings reveal that the brain mechanisms of pain modulation and reward processing, which would be associated with the PFC and the mesolimbic pathway, play a key role in chronic pain (Mitsi and Zachariou 2016).
6.1.7 Imaging/Neurological Pain Signatures
Since the earlier days of neuroimaging, researchers have kept a strong enthusiasm in finding the imaging makers for chronic pain (Box 6.1). The imaging marker, by definition, should be highly predictive of the occurrence of pain, and the absence of the marker will be predictive of the non‐occurrence of pain (Mouraux and Iannetti 2018). As shown in earlier reviews, while some brain regions showed consistent activation across the studies, many of these brain regions did not show an activation to pain selectively. Many brain regions may show an activation corresponding to the affective–motivational processing during experiments, e.g. perceiving a salient stimulus of a threat (Mouraux and Iannetti 2018). More recently, using the multivariate pattern analysis (MVPA), researchers were able to identify the assembly of regional activation that discriminated the presence of pain (Brodersen et al. 2012; Brown et al. 2011; Wager et al. 2013). The MVPA approach has been used to identify the neurologic pain signatures (NPS), i.e. ‘a multivariate brain pattern tracking nociceptive pain’ (Wager et al. 2013; Zunhammer et al. 2018). The methodological advancement greatly improved the low sensitivity/specificity of pain prediction and offered an unbiased estimation between the association of pain and brain activation because it did not pre‐select the brain regions for testing (Reddan and Wager 2018). Another recent study of MVPA reported a new signature, the stimulus intensity‐independent pain signature‐1 (SIIPS1), to predict the trial‐by‐trial pain ratings. In addition to predicting the intensity of the noxious stimulus, its response can be influenced by affective–cognitive factors of pain, which contributed to pain modulation (Woo et al. 2017).
Another issue of interest is to focus on the pattern of intrinsic functional connectivity during the resting state instead of the brain activation when patients perceive pain. The approach helps to elucidate how the ongoing and spontaneous pain disrupts one’s intrinsic functional connectivity (Kucyi and Davis 2015). The findings broaden the view of ‘neuromatrix’ from a stimuli‐based network to a resting‐state network, highlighting the dynamic fluctuation between different states in chronic pain patients (Baliki et al. 2008a; Davis et al. 2017). However, it should be noted that these ‘predictors’ – either derived from the MVPA approach or a combination of stimuli‐based and resting‐state research – did not delineate the causal relationship between pain and the neuroimaging signatures (Davis et al. 2017; Mouraux and Iannetti 2018).
6.1.8 Summary
- The concepts of pain neuromatrix highlight the multiple dimensions of pain, which correspond to an assemble of neural substrates of sensory, cognitive and emotional processing.
- Neuroimaging findings correspond to the concept of the neuromatrix by showing consistent activation in some regions across studies. However, there exists a great variation in the regions of activation.
- Neuroimaging studies on experimental pain suggest that by manipulating the cognitive–affective factors, such as the perception of threat and attention, experimenters can modulate the pain intensity perceived by subjects.
- Chronic pain may be engaged with a change in the affective–motivational network of the brain beyond the change of the sensorimotor network.
- Research on structural brain features reveals a more consistent decrease rather than an increase in GMV. The decreased GMV at the anterior insula, the cingulate cortex and the PFC have been identified in various diseases of chronic pain.
- Neuroimaging studies reveal that the brain mechanisms of pain modulation and reward processing, which would be associated with the PFC and the mesolimbic pathway, play a key role in chronic pain.
- At present, it is still difficult to delineate the causal relationship between pain and the brain solely from the neuroimaging evidence.
6.2 Chronic Pain, Neural Plasticity and Central Sensitization
6.2.1 Introduction
Chronic pain is one of the major global challenges in healthcare. It is estimated that approximately 10% of the world’s population suffer chronic pain (Goldberg and McGee 2011). Though the estimates of prevalence and incidence may vary between regions, it is of little question that chronic pain is a major medical challenge (GBD 2015 Disease and Injury Incidence and Prevalence Collaborators 2016). Moreover, chronic pain is associated with an increased disability, such as masticatory dysfunction in patients with temporomandibular disorder (TMD)‐related pain. Chronic pain has a strong negative effect on patients’ feeding behaviour and psychosocial well‐being (Nasri‐Heir et al. 2016). While acute pain is sometimes considered as ‘good pain’ because it signifies ongoing tissue damage and motivates patients to seek medical care, chronic pain exacerbates patients’ quality of life and disrupts their daily functions (Sharav and Benoliel 2008). This section outlines recent neuroimaging findings on the brain mechanisms of chronic pain. Specifically, we focus on the mechanisms of central sensitization, which have been widely studied in recent years, as a potential mechanism for persistent pain. At the neurophysiological level, central sensitization is a manifestation of the plasticity of the somatosensory nervous system (Latremoliere and Woolf 2009). We briefly discuss the role of neural plasticity in pain and behaviour in this section.
6.2.2 Definition and Classification of Chronic Pain
According to the International Classification of Diseases (ICD) version 11, chronic pain is defined as ‘persistent or recurrent pain lasting longer than 3 months’ (Treede et al. 2015; Treede et al., 2019). This general definition of chronic pain does not specify the pathological mechanisms of chronic pain, partly due to the complexity of the underlying mechanisms. The Task Force for the Classification of Chronic Pain, organized by the IASP, established the classification of chronic pain according to multiple criteria, including the aetiology of pain, the underlying pathophysiological mechanisms and the body site of pain. In the latest version of the ICD (ICD‐11), chronic pain is classified into seven major categories: (i) chronic primary pain, (ii) chronic cancer‐related pain, (iii) chronic postsurgical and posttraumatic pain, (iv) chronic neuropathic pain, (v) chronic secondary headache and orofacial pain, (vi) chronic secondary visceral pain and (vii) chronic secondary musculoskeletal pain (Treede et al. 2015). Chronic headache and orofacial pain is defined as ‘headaches or orofacial pains that occur on at least 50% of the days during at least 3 months’. It is noteworthy that inconsistency between the site of pain and the source of pain is common for chronic orofacial pain. For example, patients with neurovascular orofacial pain may perceive toothache (i.e. the site of pain) without any relevant dental lesion. The toothache derives from migraine (i.e. the source of pain) (Benoliel et al. 2010). In general, in chronic orofacial pain, the association between clinical symptoms and signs and the underlying mechanisms is not clear‐cut. Therefore, the diagnosis of chronic orofacial has long been a major challenge in dentistry.
6.2.3 Chronic Pain and Neural Plasticity
The reason why the classification and diagnosis of chronic pain are so challenging can be partly explained by the definition of pain. According to the definition by IASP, pain is associated with actual or potential tissue damage (IASP 2020). For acute pain, this association between pain and tissue damage cannot be neglected. For example, to relieve toothache, the lesions from dental pulp or periodontium need to be managed first. However, chronic pain is a long‐term experience. In addition to the identification of the tissue damage or peripheral lesions related to pain, we also need to consider how the (painful) experience is shaped and what factors contribute to the chronification of pain.
6.2.3.1 Neural Plasticity
The shaping of individual experience may be associated with the brain mechanisms of plasticity, which is generally defined as ‘the susceptibility of human behavior to modification’ at the behavioural level (Pascual‐Leone et al. 2005). In terms of neuroscience, the concept of neural plasticity specifically refers to the phenomenon that ‘neuronal and synaptic functions are capable of being moulded or shaped so that they influence subsequent perceptual experiences’ (Melzack et al. 2001). The term ‘neural plasticity’ needs further clarification. Firstly, plasticity refers to a change of the association between behaviour and the brain – not only brain functions, but also its structure can be moulded by behaviour. Secondly, these functional or structural changes should sustain when the behavioural factors are removed. For example, if persistent stimuli induce the change in the central pathway of pain processing, such a change will last for a period even when the stimuli are removed (Berlucchi and Buchtel 2009). Thirdly, because the nervous system is hierarchically organized from the cellular level (e.g. the synapse between neurons) to the whole‐brain level (e.g. the connection between brain regions), plasticity may be observed at different levels (Berlucchi and Buchtel 2009).
6.2.3.2 Brain Mechanisms of Plasticity
As shown in Chapter 2, MRI makes it feasible for researchers to assess both structural features and functional features of the brain, such as the morphometric of grey matter and the intrinsic functional connectivity, respectively. Therefore, one can identify if there exist changes in the brain under behavioural changes. In an earlier study on brain plasticity, Draganski et al. (2004) investigated the change in brain structure associated with skill training. The researchers asked subjects to practice juggling with three balls until they were skilled in juggling (Draganski et al. 2004). Structural MRI was conducted at three stages: before the training course, when they gained proficiency, and three months after the second scan. The researchers found that right after the subjects mastered juggling (i.e. at the second scan), there was increased GMV in the mid‐temporal area compared to the baseline. However, such a structural change disappeared three months after the second scan, during which period the participants have no longer practiced juggling (Draganski et al. 2004). It has been widely conceived that ‘brain structure’ is less malleable. However, the study revealed that the morphometric features still change in response to the behavioural improvement (i.e. mastering juggling) in adulthood. Secondly, such a change was not a long‐lasting one since the change in grey matter was not statistically significant once the participants stopped practicing. The finding suggests that the strength of behavioural modification (e.g. the effort of training or the intensity of an experience) may play a key role in the duration for the plastic effect to be sustained.
6.2.4 Sensitization
When the nervous system is continuously stimulated by nociceptive stimuli, the nervous system may be sensitized by persistent stimulation. Sensitization is defined by the IASP as ‘increased responsiveness of nociceptive neurons to their normal input, and/or recruitment of a response to normally subthreshold inputs’ (IASP 2020) (Figure 6.3). Pain sensitization is closely associated with neural plasticity, especially at the neuronal level (Woolf and Salter 2000). As discussed in the following sections, chronic pain does not fully correspond to the intensity of nociceptive stimulation. In the patients, a gain of pain (e.g. hyperalgesia) may be associated with sensitization of the peripheral and the CNS, which reflect plastic changes in the neural pathway of pain processing (Woolf and Salter 2000).
6.2.4.1 Peripheral vs. Central Sensitization
In normal status, information from noxious stimuli and non‐noxious stimuli, such as a tactile stimulus, is transduced by Aδ and Aβ fibres, respectively. The information is relayed to the CNS neuron in the spinal cord or the brainstem for the processing of nociception and tactile sensation (Figure 6.3a). Sensitization may occur at both peripheral and central sites. For example, when inflammation occurs at the peripheral sites (e.g. the apical lesion of apical periodontitis), inflammatory mediators (e.g. PGE2 and bradykinin) (Woolf and Salter 2000) may reduce the threshold of the peripheral terminals of nociceptors, rendering them more sensitive to a noxious stimulus. Under the sensitized condition (Figure 6.3b), neurons would show increased responsiveness to noxious stimuli compared to the non‐sensitized condition (Figure 6.3a). Therefore, peripheral sensitization may be associated with hyperalgesia, i.e. ‘increased pain from a stimulus that normally provokes pain’ (IASP 2020). Sensitization may occur at the central site (e.g. the spinal cord or the brainstem). Central sensitization is defined by the IASP as ‘increased responsiveness of nociceptive neurons in the central nervous system to their normal or subthreshold afferent input’ (IASP 2020). In the case of central sensitization, persistent stimulation to the central nervous system (CNS) neurons would amplify their signals (Figure 6.3c). Such an amplification occurs when an excitatory synapse is facilitated or an inhibitory synapse is depressed (Woolf and Salter 2000). Therefore, the sensitization of the nervous system is associated with alterations in the experience of pain.

Figure 6.3 Mechanisms of peripheral and central sensitization. (a) In the normal status, signals induced by noxious stimuli and non‐noxious (e.g. tactile) stimuli are transduced via the pathway of nociceptive and tactile processing, respectively. (b) In peripheral sensitization, neurons would show increased responsiveness to noxious stimuli. For example, the inflammation at the peripheral site (i.e. the light red area) may reduce the threshold to evoke a response. The signals for subsequent nociceptive processing are amplified. Therefore, peripheral sensitization may be associated with hyperalgesia. (c) In secondary hyperalgesia (in contrast to primary hyperalgesia (b)), noxious stimulation to the area surrounding the site of injury or inflammation (i.e. the black arrow) elicits an amplified pain. The amplification is mediated by the central neurons (i.e. the red circle), which have been sensitized by constantly receiving nociceptive inputs from the primary lesion (i.e. the circled light grey area). (d) In allodynia, non‐noxious tactile stimuli are conveyed by the Aβ fibre elicit pain. Note that at the central level, both the nociceptive and tactile pathways converge on central nociceptive neurons. The central neurons (the red square) have been sensitized by constantly receiving nociceptive inputs from the primary lesion.
6.2.4.2 Allodynia
Until now, most of our understanding of central sensitization has been based on animal research. A key feature of central sensitization is the amplification of the neuronal responses to stimuli, which can be noxious or non‐noxious. The condition that pain is perceived ‘due to a stimulus that does not normally provoke pain’ is termed allodynia (IASP 2020). Some forms of allodynia, such as ‘thermal allodynia’, derive from changes at the peripheral level (Sharav and Benoliel 2008). However, the symptom of mechanical allodynia should be carefully examined because it may indicate central sensitization. Mechanical allodynia is observed when a non‐noxious stimulus (e.g. cotton‐swapping), which normally induces Aβ fibre (for tactile sensation), evokes pain. In normal status, signals from Aβ fibre do not excite the CNS neuron of nociceptive processing, which has a higher threshold of response (Figure 6.3a). However, in central sensitization, the CNS neuron of nociceptive processing may respond to subthreshold afferent input. As shown in Figure 6.3d, in the condition of central sensitization, the signals conveyed by the Aβ fibre may excite the nociceptive neurons in the CNS lead to pain. Therefore, the experience of mechanical allodynia is also termed as ‘Aβ pain’ (Sharav and Benoliel 2008). It is noteworthy that beyond the spinal cord and the brainstem, sensitization may occur at a higher level in the CNS. For example, in patients with neuropathic pain, the thalamus showed a high rate of spontaneous firing (Lenz et al. 1989). As discussed in Section 6.3, the neuropathic pain of orofacial regions may be associated with central sensitization of the trigeminal pathway from the brainstem to the thalamus (Park et al. 2006).
6.2.4.3 Hyperalgesia
Hyperalgesia can be differentiated into primary and secondary hyperalgesia according to the relationship between the site of tissue damage and pain (Johanek et al. 2006). Primary hyperalgesia occurs at the same site where damage occurs (Schmidt and Willis 2007). In contrast, secondary hyperalgesia develops when noxious stimuli are applied in the area surrounding the site of tissue damage (Figure 6.3c). Secondary hyperalgesia is associated with central sensitization and is commonly reported by patients with chronic pain. Increased pain is mediated by the CNS neurons that are sensitized due to continuous nociceptive inputs from the site of primary hyperalgesia (Schmidt and Willis 2007).
6.2.5 Neuroimaging Research on Central Sensitization
As a critical mechanism of chronic pain, central sensitization has been a widely investigated topic of neuroimaging research in recent years. The recent findings of pain sensitization from experimental research (i.e. pain sensitization induced by experimental methods) or clinical research (i.e. chronic pain associated with sensitization) are summarized in Table 6.2.
6.2.5.1 Research on Sensitization Induced by Experimental Methods
For healthy subjects, central sensitization can be studied using the model of secondary hyperalgesia, as noted above, which refers to the pain derived from stimuli in the area surrounding the site of an injury or inflammation. Secondary hyperalgesia can be induced by chemical stimuli (e.g. intradermal capsaicin) (Liang et al. 2016) or continuously electrical stimuli (Van Den Broeke et al. 2017). If secondary hyperalgesia is associated with changes at the central level, one may expect that the pharmacological intervention that modulates the CNS activity would reduce the hyperalgesia. This hypothesis is confirmed by Iannetti et al. (2005), who demonstrated that gabapentin, which has a modulatory effect on CNS activity, showed an antihyperalgesic effect for secondary hyperalgesia. Using the model of sensitization by thermal stimulation, Hansen et al. (2018) found that in healthy subjects the size of secondary hyperalgesia was not associated with the volume of brain regions of pain processing. Instead, the increased size of secondary hyperalgesia was associated with changes in functional connectivity of the sensorimotor network and the DMN (Hansen et al. 2019). Using electroencephalography (EEG), researchers also identified the neural activity associated with secondary hyperalgesia when subjects were receiving mechanical or electrical stimuli (Liang et al. 2016; Van Den Broeke et al. 2017; Van Den Broeke et al. 2015). For example, healthy subjects received intradermal capsaicin to induce an area of secondary hyperalgesia. Mechanical stimuli (i.e. pinprick stimuli) were applied to the area to elicit secondary hyperalgesia. After capsaicin injection, EEG also revealed the pinprick‐evoked potentials, which may reflect pain processing associated with central sensitization (Van Den Broeke et al. 2015). A recent study revealed that hypersensitivity induced by mechanical stimuli may be less pronounced when subjects were engaged with a cognitive–demanding task, suggesting a modulatory effect of cognitive processing on sensitization (Torta et al. 2020). In addition to mechanical stimuli, prolonged pain can also be elicited by heat stimuli with the induction of capsaicin. The pain intensity was correlated with the EEG signatures of the pain‐free period preceding pain induction (Furman et al. 2018). Heat stimuli were also used to induce hyperalgesia, as shown in the experimental model of first‐degree burn injury (Asghar et al. 2015).
Table 6.2 Neuroimaging research on brain mechanisms of central sensitization and pain (since 2015).
Source | Participants | Methods |
---|---|---|
Experimental pain | ||
Torta et al. (2020) | 60 healthy adults, secondary hyperalgesia induced by low‐frequency electrical stimulation of the skin | EEG/mechanical pinprick stimuli and cognitive tasks of increasing difficulty |
Hansen et al. (2019) | 115 healthy adults, secondary hyperalgesia induced by thermal sensitization | rs‐fMRI/functional connectivity |
Hansen et al. (2018) | 121 healthy adults, secondary hyperalgesia induced by thermal sensitization | sMRI/FreeSurfer |
Furman et al. (2018) | 44 healthy adults, prolonged pain induced by capsaicin‐heat pain | EEG before and after capsaicin incubation/heat stimuli |
Van Den Broeke et al. (2017) | 16 healthy adults, secondary hyperalgesia induced by HFS | EEG before and 20 min after HFS/pinprick stimuli |
Liang et al. (2016) | 12 healthy adults, hyperalgesia induced by intraepidermal injection of capsaicin | EEG/intraepidermal electrical stimulation |
Van Den Broeke et al. (2015) | 19 healthy adults, secondary hyperalgesia induced by intradermal capsaicin | EEG before and 30 min after capsaicin injection/pinprick stimuli |
Asghar et al. (2015) | 40 healthy adults, secondary hyperalgesia induced by a first‐degree burn injury | sMRI, fMRI before and after induction/mechanical noxious stimuli |
Disease‐related pain: headache | ||
Chong et al. (2020) | 18 patients with cluster headache and 19 patients with migraine (22 healthy controls) | sMRI/FreeSurfer |
Aguila et al. (2016) | 20 patients with migraine (20 healthy controls) | MRS |
Becerra et al. (2016) | 32 patients with migraine (33 healthy controls) | 1H‐MRS |
Chen et al. (2015) | 15/19 patients of migraine w/wo cutaneous allodynia (20 healthy controls) | fMRI/transcutaneous electrical nerve stimulation at the forearm |
Chen et al. (2017) | 27 patients with medication‐overuse headache (27 normal controls) | sMRI/volumetric analysis |
Disease‐related pain: fibromyalgia | ||
Hazra et al. (2020) | 50 patients of fibromyalgia (50 healthy controls) | fNIRS/cold pressure test |
Kaplan et al. (2020) | 27/27 patients with rheumatoid arthritis w/wo concomitant fibromyalgia | fMRI |
Hadanny et al. (2018) | 30 patients with fibromyalgia syndrome and a history of childhood sexual abuse receiving hyperbaric oxygen therapy and psychotherapy | dMRI, SPECT |
Bosma et al. (2016) | 14 patients with fibromyalgia syndrome (15 pain‐free controls) | fMRI/heat pain stimuli |
Lim et al. (2015) | 17 patients with fibromyalgia (21 healthy controls) | MEG/median nerve stimulation at the wrist |
Disease‐related pain: chronic back/joint pain | ||
Yu et al. (2020) | 90 patients with chronic low back pain (74 healthy controls) | rs‐fMRI at baseline and after manipulation for pain |
Malfliet et al. (2019) | 94 patients with chronic spinal pain | sMRI/FreeSurfer |
De Groote et al. (2020) | 10 patients with failed back surgery syndrome | rs‐fMRI/at baseline, 1 month and 3 months after HF‐SCS |
Nishioka et al. (2019) | 10 patients with painful legs and moving toes syndrome (34 healthy controls) | MRI, SPECT |
Soni et al. (2019) | 24 patients of knee osteoarthritis receiving arthroplasty, 10 with nociceptive pain and 14 with neuropathic‐like pain according to PainDETECT | fMRI/punctuate and cold stimuli |
De Kruijf et al. (2016) | 1191 patients with chronic joint pain | sMRI/FreeSurfer |
Other disease‐related pain | ||
Karafin et al. (2019) | 15 patients with sickle cell disease (SCD) and chronic pain and 7 SCD patients without chronic pain (10 healthy controls) | rs‐fMRI/functional connectivity |
Zempsky et al. (2017) | SCD patients (healthy controls) | rs‐fMRI, fMRI/painful stimuli |
Böttcher et al. (2019) | 19 patients with primary dysmenorrhea (20 healthy controls) | fMRI/painful rectal distensions |
Liu et al. (2017) | 41 patients with primary dysmenorrhea (41 healthy controls) | dMRI/tractography |
Gupta et al. (2015) | 29 patients with localized provoked vulvodynia and 29 patients with irritable bowel syndrome (29 healthy controls) | rs‐fMRI/functional connectivity |
Coppieters et al. (2018) | 37 patients with chronic whiplash‐associated disorders and 37 patients with chronic idiopathic neck pain (31 healthy controls) | sMRI, dMRI |
Coppieters et al. (2017) | 31 patients with chronic whiplash‐associated disorders and 34 patients with chronic idiopathic neck pain (28 healthy controls) | sMRI/FreeSurfer |
Notes: dMRI: diffusion magnetic resonance imaging; EEG: electroencephalography; fMRI: functional magnetic resonance imaging; fNIRS: functional near‐infrared spectroscopy; HFS: high‐frequency electrical stimulation; HF‐SCS: high‐frequency spinal cord stimulation; MEG: magnetoencephalography; MRI: magnetic resonance imaging; MRS: magnetic resonance spectroscopy; rs‐fMRI: resting‐state functional magnetic resonance imaging; SPECT: single photon emission computed tomography; sMRI: structural magnetic resonance imaging.
6.2.5.2 Research on Patients with Chronic Pain
In addition to the experimental model, central sensitization has also been investigated for a variety of chronic pain, including fibromyalgia (Bosma et al. 2016; Hazra et al. 2020; Kaplan et al. 2020; Lim et al. 2015), migraine (Aguila et al. 2016; Becerra et al. 2016; Chong et al. 2020), osteoarthritis (Soni et al. 2019), dysmenorrhea (Böttcher et al. 2019; Liu et al. 2017), whiplash pain (Coppieters et al. 2017; Coppieters et al. 2018) and chronic pain with motor syndrome (Nishioka et al. 2019). Most of the studies made a comparison between a clinical group and a group of non‐painful healthy controls (Table 6.2). A variety of brain features were investigated, including the morphology of grey matter, the morphology of white matter, functional connectivity and structural connectivity. However, the neuroimaging studies do not show very consistent findings. For example, Chen et al. (2017) investigated the patients with migraine and medical‐overuse headache and found an increased thalamic volume in patients compared to controls. De Kruijf et al. (2016) investigated GMV of community‐dwelling subjects. They found that in female subjects individuals with chronic joint pain showed a smaller GMV at the hippocampus, the temporal and the frontal lobes (De Kruijf et al. 2016). The diversity of results may be largely attributed to the different pathological mechanisms of chronic pain. In terms of functional connectivity, an alteration in the DMN and the salience network is consistently reported in several studies (De Groote et al. 2020; Gupta et al. 2015; Karafin et al. 2019; Zempsky et al. 2017). Moreover, a recent study on patients with chronic low back pain revealed that deficient mesocorticolimbic connectivity, which may signify dysfunctions reward network, may play a key role in increased pain sensitivity (Yu et al. 2020). In patients with chronic spinal pain, the features of grey matter morphology are significantly correlated with the emotional representations, chronicity and pain catastrophizing of female patients (Malfliet et al. 2019). Therefore, the diversity of brain mechanisms identified in these studies may reflect the difference in cognitive–affective processing of pain.
It should be noted that in most of the studies a direct comparison between the clinical and the control groups was made to disclose the difference in the brain mechanisms associated with central sensitization. However, these differences may not be fully attributed to central sensitization. Because clinical symptoms (e.g. mechanical allodynia) are highly associated with central sensitization, it is critical to investigate the association between the clinical symptoms and the brain on an individual basis. For example, Chen et al. (2015) reported that the patients with allodynia showed reduced activation of the inferior parietal lobule compared to those without allodynia. Soni et al. (2019) assessed preoperative neuropathic‐like pain of patients with knee osteoarthritis using a standard questionnaire. They found that higher neural activity in the rostral ventromedial medulla (RVM), a region known for pain modulation, was associated with moderate‐to‐severe pain after arthroplasty (Soni et al. 2019). In another study, Aguila et al. (2016) investigated the central sensitization of migraine patients using standard questionnaires. They assessed the level of glutamate and γ‐aminobutyric acid (GABA), a critical modulator of pain, using magnetic resonance spectroscopy (MRS). They reported a positive association between the GABA levels and the central sensitization scores (Aguila et al. 2016). In sum, neuroimaging findings have disclosed a complicated pattern of the brain mechanisms related to chronic pain. To affirm the role of central sensitization in chronic pain, the association between the brain and the clinical symptoms of pain sensitization would require further investigation.
6.2.6 Summary
- The classification of chronic pain is generally based on multiple criteria, including the aetiology of pain, the underlying pathophysiological mechanisms and the body site of pain.
- For patients with chronic pain, clinicians need to consider not only the association between pain and tissue damage but also the cognitive–affective factors that contribute to the chronic experience of pain.
- Sensitization may occur at the peripheral or the central level (e.g. the spinal cord or the brainstem). Central sensitization is defined by the IASP as ‘increased responsiveness of nociceptive neurons in the central nervous system to their normal or subthreshold afferent input’.
- The symptom of mechanical allodynia should be carefully examined because it may indicate central sensitization. Secondary hyperalgesia develops in the area surrounding the injury, which is also associated with central sensitization.
- Neuroimaging findings have disclosed a complicated pattern of the brain mechanisms related to chronic pain. To affirm the role of central sensitization in chronic pain, the association between the brain and the clinical symptoms of pain sensitization would require further investigation.
6.3 Brain Mechanisms of Chronic Orofacial Pain
6.3.1 Introduction
As outlined in the preceding sections, chronic orofacial pain is detrimental to individual well‐being and therefore poses a great challenge in healthcare. It is estimated that in the US, 5% of adults have reported chronic pain in the face or jaw during a 3‐month period (Benoliel et al. 2019). According to the latest classification by the ICD‐11, orofacial pain is categorized into the ‘primary’ or ‘secondary’ domain. Chronic primary orofacial pain includes chronic primary TMD pains, chronic burning mouth and pain with an idiopathic origin (e.g. persistent idiopathic dentoalveolar pain). Chronic secondary orofacial pain includes chronic dental pain (derived from pulpal or periapical lesions) and chronic neuropathic orofacial pain (derived from lesions or diseases related to the trigeminal nerve system) (Benoliel et al. 2019). It is noteworthy that this classification, just like the current classification of chronic pain in general, does not reflect a clear association between clinical symptoms and pathological mechanisms (Treede et al. 2015, 2019). For dental pain with an intraoral lesion (e.g. pulpitis or periodontitis), treating the lesion would predict an improvement of clinical symptoms. In contrast, pain related to TMD or trigeminal neuropathy shows a great diversity in clinical symptoms in terms of individual variability in sensory and emotional experiences (Nasri‐Heir et al. 2016). Since pain is a multi‐dimensional construct related to cognitive–affective processing (see Section 6.1), the role of our brain in the clinical diversity of chronic orofacial pain should not be neglected. This section focuses on the recent neuroimaging findings on the brain mechanisms of chronic orofacial pain. Particularly, we focus on the potential brain mechanisms of pain related to TMD and trigeminal neuropathy, two major challenges in the clinical management of orofacial pain.
6.3.2 TMD‐related Pain
Pain is one of the major chief complaints reported by patients with TMD. Moreover, in the patients, pain is usually associated with functional limitations, such as a reduced amount of maximal mouth opening. Therefore, TMD has a negative impact on patients’ quality of life, not only for pain but also for dysfunctions for feeding. There has been a large body of neuroimaging evidence on the brain mechanisms of TMD‐related pain. However, inconsistent findings have been reported, partly because of the pathological complexity and great inter‐individual heterogeneity in clinical symptoms and signs. The neuroimaging findings from recent studies are summarized in the following sections.
6.3.2.1 Findings from Meta‐analytic Studies
Animal research has identified the role of the thalamocortical system in pain processing. It has been conceived that the lateral and the medial pathways, respectively, take part in the processing of sensory–discriminative information and the emotional‐motivational information of pain (Groh et al. 2018). Therefore, the earlier neuroimaging studies focused on structural and functional features related to the thalamocortical system. A general hypothesis is that compared to healthy controls patients with TMD‐related pain would show alterations in the brain regions associated with the thalamus and the somatosensory cortices, which have been identified in various neuroimaging studies of acute pain (see Section 6.1). However, the meta‐analytic findings from neuroimaging studies of TMD‐related pain reveal a different story. An earlier meta‐analytic study (Lin 2014
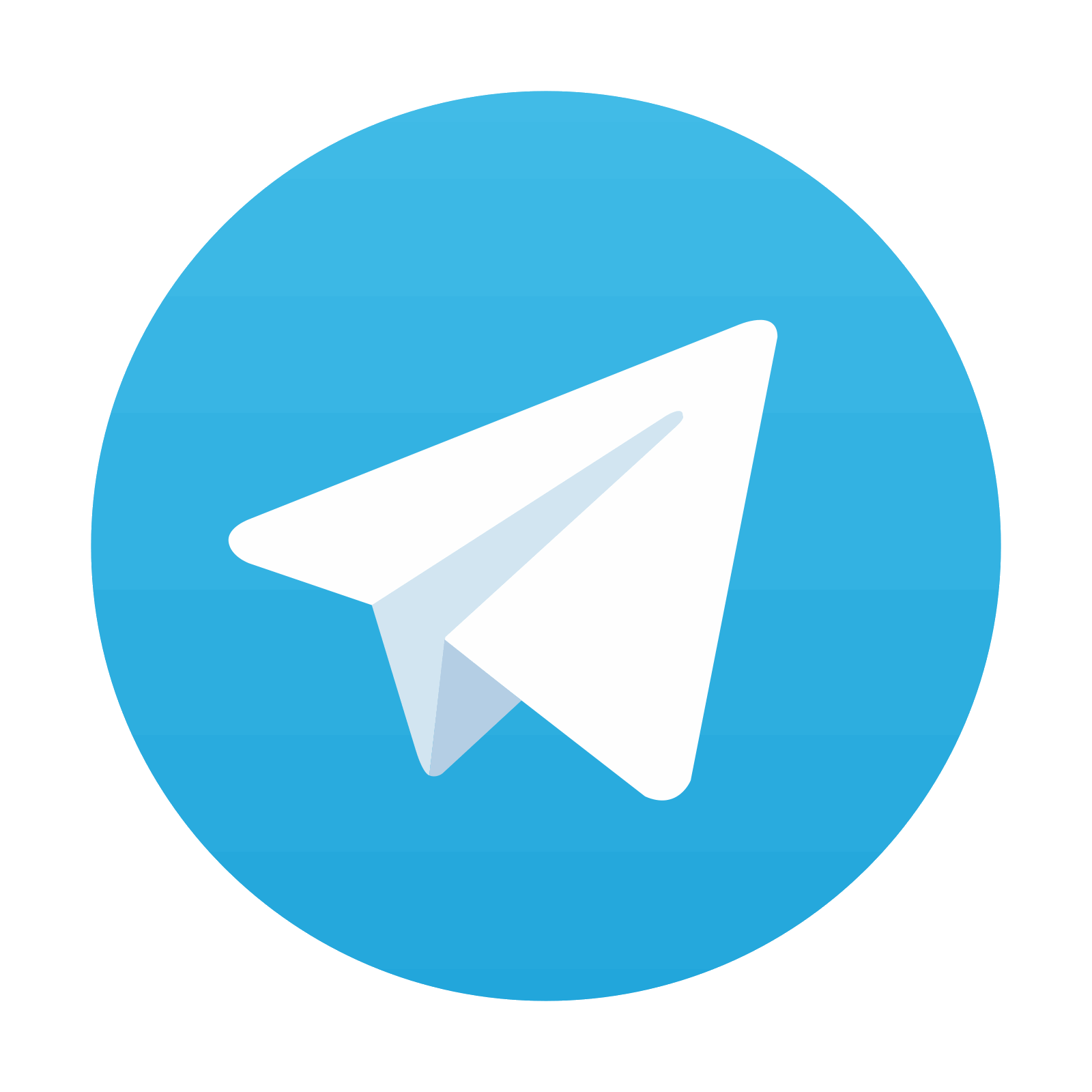
Stay updated, free dental videos. Join our Telegram channel

VIDEdental - Online dental courses
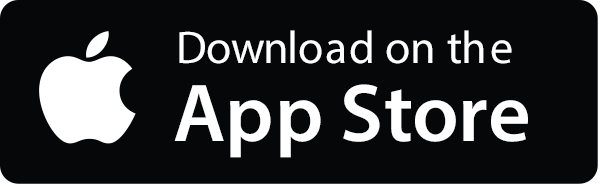
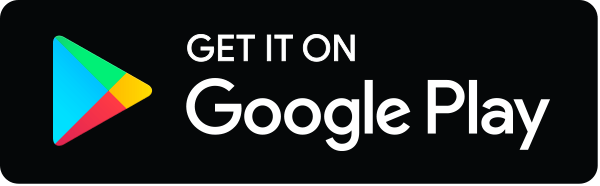