Fig. 6.1
Comparison of EQV magnitude within (a) cortical bone and (b) cancellous bone for both surgical approaches at different load directions
Figure 6.2 shows the stresses were uniformly distributed in the zygoma in two main directions, towards the temporal and frontal processes. Stresses were more gradually and largely distributed at the maxillary sinus lateral wall for extramaxillary as compared to intrasinus approach. The stresses were also tended to be generated over orbital floors and infratemporal fossae region. A wider stress concentration region was developed at the alveolar ridge bone which was then predominantly transferred towards the palatal area and infrazygomatic crest region in the bucco-lingual side, at surrounding maxilla region when the models were loaded by oblique forces.
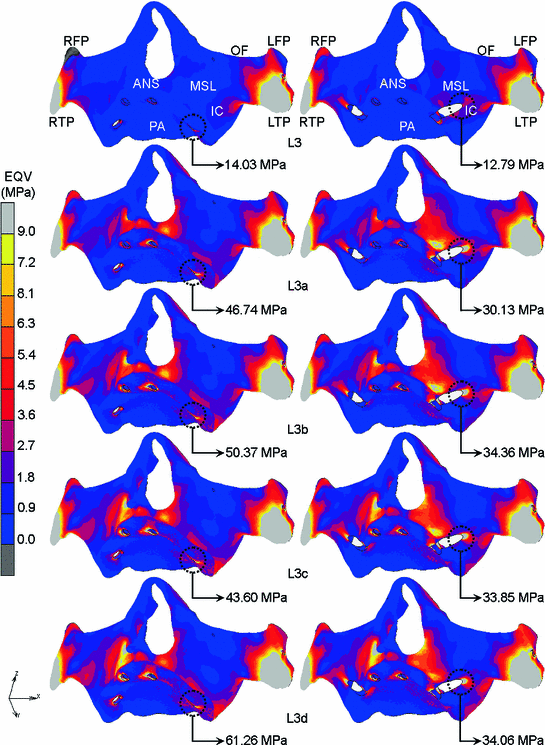
Fig. 6.2
Comparison of EQV distribution within cortical bone under L3 to L3d for the intrasinus and extramaxillary approach (left to right) as viewed from frontal (ANS Anterior nasal spine, IC Infrazygomatic crest, LFP Left frontal process, LTP Left temporal process, MSL Maxillary sinus lateral wall, OF Orbital floor, PA Palatal area, RFP Right frontal process, RTP Right temporal process)
Regarding the EQV pattern at the maxillopterygoid area, high stresses (14.03–61.26 MPa) were mainly concentrated at the alveolar crest region around the neck of implant body in intrasinus approach. The presence of opening path for the implant placement at the crestal region adjacent to the applied loading location in intrasinus approach increased the strength of alveolar bone to retain the implant position, thus, resulting in high stress concentration. For the extramaxillary approach, the location of opening path for implant insertion was in the second premolar region, which was further away from the loading point. This resulted in an evenly distributed stress within the bone around the first molar.
The high stresses generated could also be associated to the influence of total contact area between implant body and bone tissues. According to Javed et al., the threaded implant design could increase the primary stability by reducing the micromovement of implant [1, 2]. The zygomatic implant used in intrasinus approach is likely to have a high contact area as the implant surface area increases due to the thread along the implant body. However, the threaded part was ignored through the preparation of FEA models and has been simulated via friction coefficient in contact properties modelling to represent a strong attachment to bones (see Sect. 4.5.2). The implant-bone contact had occurred at the alveolar ridge, slightly in the palatal aspect and at the jugale point region of zygoma, which has resulted in a smaller mating surface than that of extramaxillary approach. The percentage of bone-implant contact area for the extramaxillary approach was higher by the placement of implant body externally to the maxilla particularly outside the maxillary sinus wall and end up in the zygoma (see Sect. 4.6). It is interesting to note that the coronal part of implant body had no threads to avoid infections of the soft tissue [3]. However, the insertion path of zygomatic implant had increased the contact area of implant body to bone and therefore reduced the level of stress within the bones.
The findings, however, found that areas surrounding the anterior nasal spine and the maxillary sinus wall in extramaxillary approach, sustained more stresses as compared to the one in intrasinus approach with largely stress widespread due to the instability of implant body and the path of load transfer. More stresses localised (12.79–34.36 MPa) at the edges of the maxilla relevant to the coronal part of implant body. The force passed through the framework, soft tissue, zygomatic implant body and then widely dissipated in the maxilla and the surrounding areas. The high bone stress at the maxillary edges around the coronal portion of implant body might be due to large rotational effects from implant body caused by torque generated. As the distance of zygomatic implant head to the loading point (1st molar region) is longer than those in the intrasinus approach, the magnitude of torque produced increases proportionally, leaving behind a high stress level on the surrounding bone. Another possible explanation for this is that the existence of sharp edges of the maxilla relative to the placement of implant. The high stress value in the maxilla is a concern because it could lead to bone loss or resorption in a long-term treatment [4–10]. It is important to prevent overloading since it could make bone more fragile by destructing implant-bone osseointegration and lead to fatigue failure of the implant [6, 11].
A higher density of cortical bone of the maxilla compared to cancellous bone (see Sect. 2.1) is the factor of high magnitudes of stress produced, which provides better resistance to deformation [4, 12]. Based on literature, the mechanical stresses started to be generated and concentrated when two materials with different properties (Young’s modulus) had contacted each other after they were loaded [8, 13]. In this numerical simulation, the first part that the implant (high modulus of elasticity) met with is soft tissue (low modulus of elasticity), however, there was no significant result of stress observed. The next layer that contacted with the implant was the crestal region of cortical bone that having higher modulus of elasticity than cancellous bone and soft tissue. It absorbed most of the stresses transferred from the implant body [8, 12, 14, 15].
Most of the stresses produced from simulated occlusal loading were sustained by the zygoma and appeared to be less dependent on the maxillary anchorage. These results were in agreement with the findings of Ujigawa et al. who reported that the applied loadings were transferred through the infra-zygomatic crest and directed into the temporal and frontal processes of zygomatic bone [16]. In a study done by Yushino et al. it was also agreed that the masticatory loading was primarily transmitted into the zygomatic bone [17]. Furthermore, the present findings seem to be consistent with other researches that the zygoma was claimed to absorb most of the posterior loads [18, 19]. The occlusal forces were dissipated peripherally to the surrounding bony structures through three major trajectories; maxillonasal, maxillozygomatic and maxillopterygoid [20]. The stress dispersion to the orbital floor around the lacrimal bone and the frontal process was also confirmed, however, it was just in minimal.
The magnitude of maximum stress generated within the cortical layer of zygomatic bone for both intrasinus and extramaxillary approaches exceeded the yield strength of cortical bone, 69 MPa [21]. However, the percentage of nodal stress did not cover a large volume that could result in bone failure. The percentage of nodal stress higher than 69 MPa was only about 0.1 % for both surgical approaches.
The numerical results of the analysis showed that vertical and oblique loads resulted in different stress and displacement patterns within all models for the intrasinus and extramaxillary approach. The role of horizontal component in oblique load cannot be compromised since it has resulted in greater stress values and concentration within the bones specifically around the implant body than the pure vertical load. There was evidence where the bone remodelling has a strong correlation with the generated equivalent stresses. As discussed by Barbier et al., the horizontal component plays a major role in determining the difference degree of bone remodelling compared to axial loads. Therefore, the effect of horizontal load should be avoided because the excessive load could induce the progressive stresses increase and end up with the mechanical failure risk of implants. In general, the oblique load effect is approximately two times higher than the vertical load by considering the mean value of EQV generated within all models tested in this analysis.
6.1.2 Mechanical Stress Distribution Within the Framework
Also, in general, the application of oblique loads had influenced a higher stress value within the framework as compared to the vertical load in both approaches as shown in Fig. 6.3. Particularly, the framework model in intrasinus approach seemed to record a higher percentage of EQV increase than extramaxillary when it was obliquely loaded, which is about 43, while extramaxillary was only recorded about 8 % increase. As the force inclination increased, the magnitudes of EQV will increase, too. In comparison, extramaxillary approach showed a higher EQV level than intrasinus with 12 % increase when vertical load was applied at similar load location. A larger stress dispersion region was created when the model exerted by oblique loads which strongly concentrated at the framework-implant connection in both surgical approaches. The developed stress concentration area was seemed to become smaller as the angle of force components increased respected to the implant axis. This situation was observed in both intrasinus and extramaxillary approaches as the widest stress intensity region was found under L3a (Fig. 6.4).
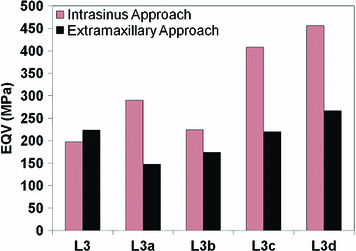
Fig. 6.3
Comparison of EQV magnitude within the framework for both surgical approaches
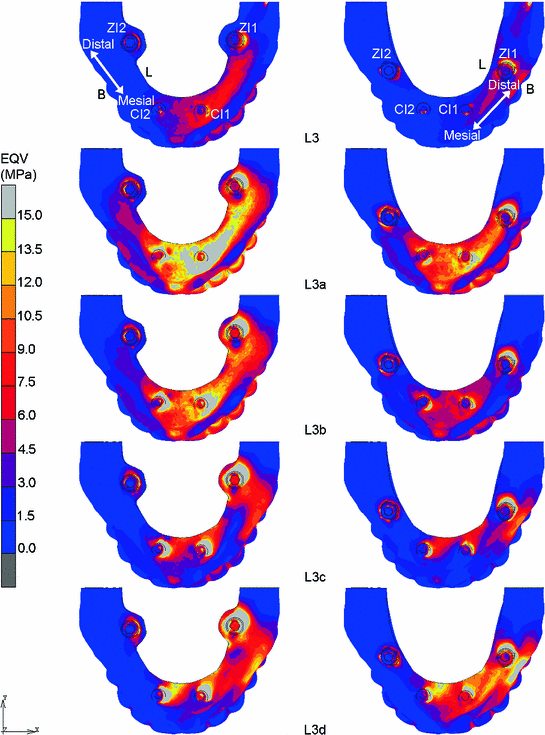
Fig. 6.4
Comparison of EQV distribution within framework under L3 to L3d for the intrasinus and extramaxillary approach (left to right) as viewed from top axial (B = Buccal, L = Lingual)
6.1.3 Mechanical Stress Distribution Within the Implants
As illustrated in Figs. 6.5a, b and 6.6a, when the results of EQV within the implants are examined generally, it is observed that the stress values were significantly decreased as the angle of load increased as shown in both intrasinus and extramaxillary approaches, however, the stresses were slightly increased back under L3d. In contrast, the stress values within CI2 on the non-working side were kept increased proportional to loading inclinations, as illustrated in Fig. 6.6b. Concerning the different load directions, the highest EQV was recorded under L3a for both zygomatic implants with intrasinus exhibited 28 and 7 % higher stress than extramaxillary approach in ZI1 and ZI2, respectively. As expected, ZI1 model located on the working side possessed a higher stress value than ZI2 (13–76 %). Also, in ZI1 model, the maximum stress that generated under L3a was increased about 21 and 7 % for intrasinus and extramaxillary approach, respectively, as compared to the vertical load at similar point of load application.
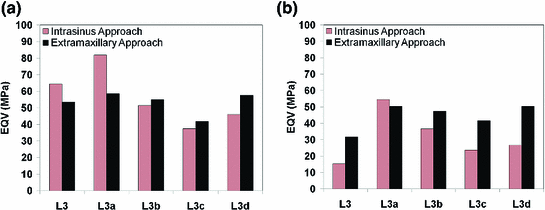
Fig. 6.5
Comparison of EQV magnitude within (a) ZI1 (working side) and (b) ZI2 (non-working side) for both surgical approaches at different load directions
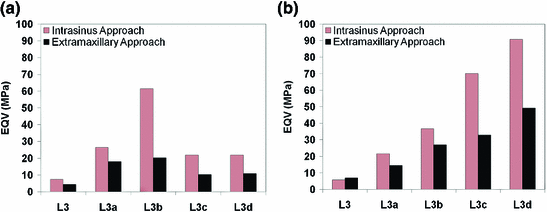
Fig. 6.6
Comparison of EQV magnitude within (a) CI1 (working side) and (b) CI2 (non-working side) for both surgical approaches at different load directions
For the conventional implants, intrasinus approach seemed to record higher EQV magnitudes than extramaxillary approach within both CI1 (32–67) and CI2 (26–53 %). A wider stress distribution area was seen at the coronal portion of implant bodies as the load inclination increased (Figs. 6.7, 6.8, 6.9, 6.10).
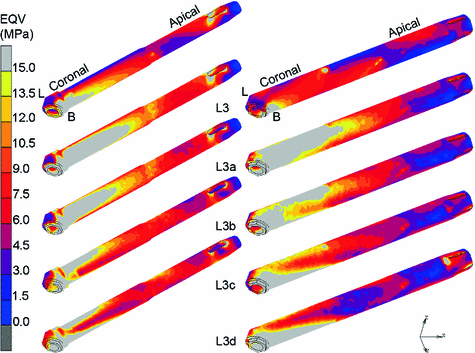
Fig. 6.7
Comparison of EQV distribution within ZI1 under L3 to L3d for the intrasinus and extramaxillary approach (left to right) as viewed from frontal
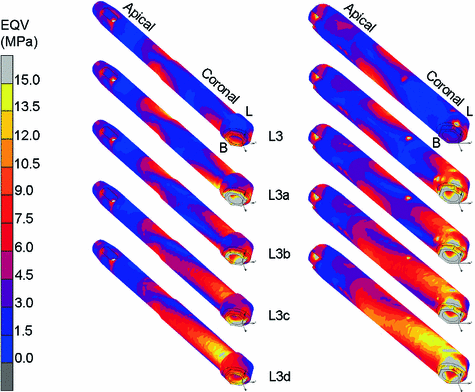
Fig. 6.8
Comparison of EQV distribution within ZI2 under L3 to L3d for the intrasinus and extramaxillary approach (left to right) as viewed from frontal
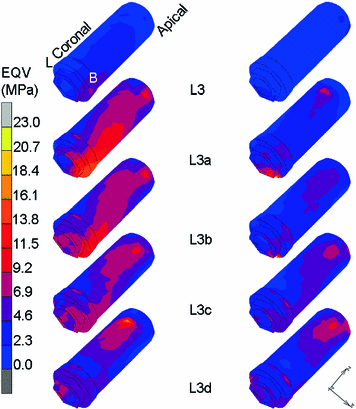
Fig. 6.9
Comparison of EQV distribution within CI1 under L3 to L3d for the intrasinus and extramaxillary (left to right) as viewed from frontal
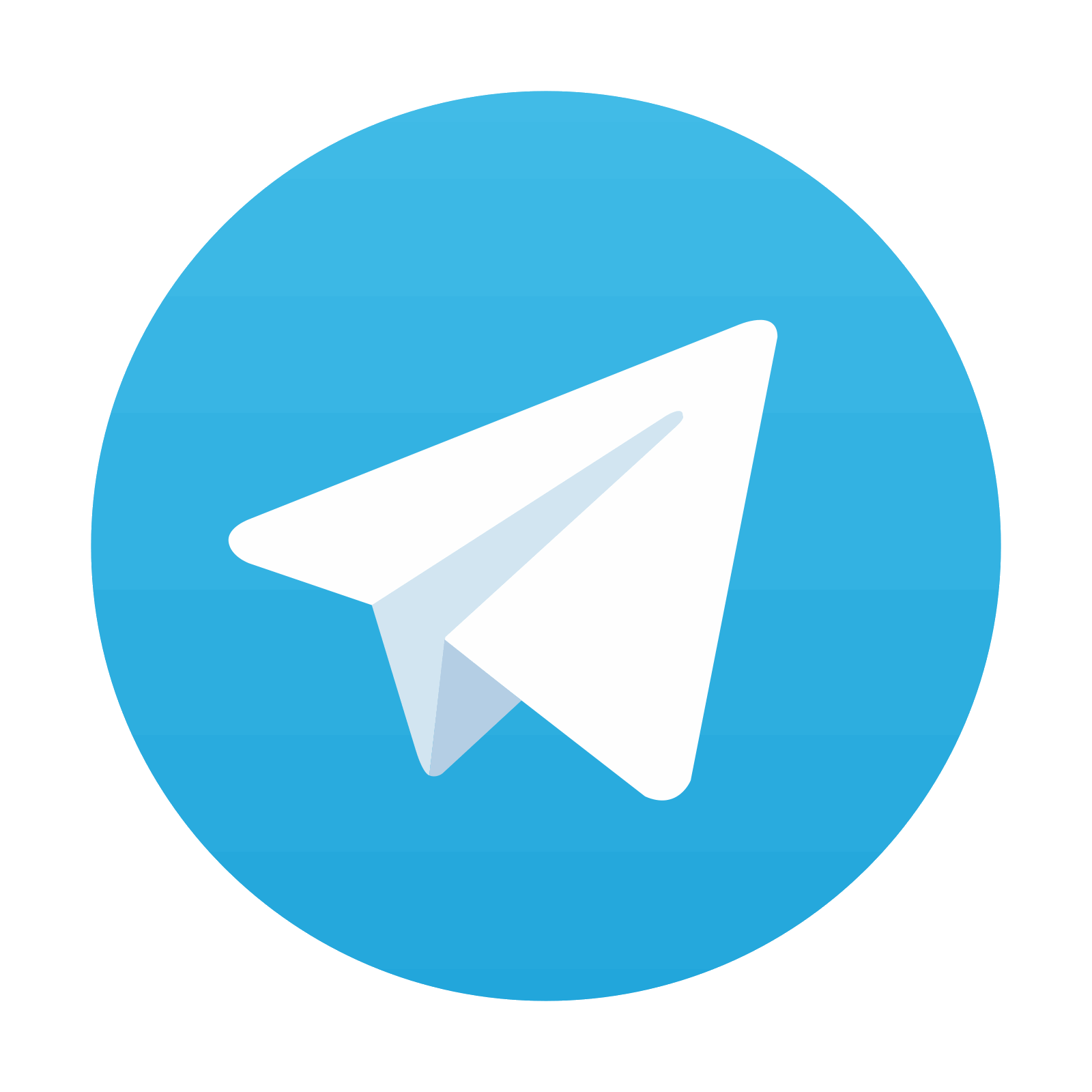
Stay updated, free dental videos. Join our Telegram channel

VIDEdental - Online dental courses
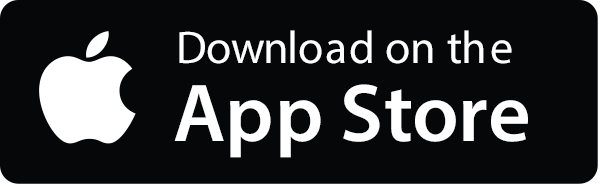
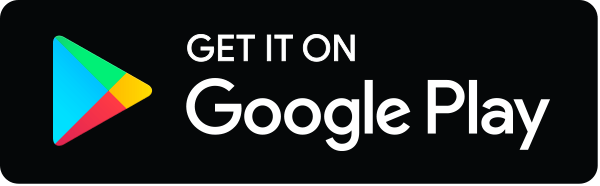