2
Lasers and Wound Healing
Georgios E. Romanos
Stony Brook University, School of Dental Medicine, Stony Brook, NY, USA
2.1 Introduction
The alterations of living tissue after the use of laser irradiation are of particular importance for the surgically active clinician. Various investigations still try today to explain the influence of the laser light on wound healing. The use of low‐level‐therapy (low intensity) lasers (soft lasers) possibly provides biostimulatory effects in the tissues (biomodulation), while the various wavelengths and features of surgical lasers (hard lasers) influence wound healing directly or indirectly.
A less well‐known clinical application is the therapeutic use of low‐dose biophotonics, termed photobiomodulation (PBM) therapy, which is aimed at alleviating pain and inflammation, modulating immune responses, and promoting wound healing and tissue regeneration. There are potential paths for clinical translation with PBM therapy with an emphasis on craniofacial wound healing and a novel opportunity to examine fundamental nonvisual photobiological processes as well as develop innovative clinical therapies in order to control or eliminate conventional invasive procedures (Arany 2016). Although, expert opinions based on ongoing research studies and reported literature are offered, and noninvasive, economical, and multipurpose light devices are attractive tools for wound management, there is an urgent need in the wound care community to develop optimal clinical protocols for use based on well‐designed, rigorous clinical research studies (Mosca et al. 2019).
Dependent on the laser wavelength, power settings, irradiation period, fluence, and the application technique, the light will absorb or scatter within the tissue accelerating or delaying wound healing, and based on the host response, the heat transfer will create positive of negative effects on the target tissues. All these parameters can have an impact on the final clinical outcome.
More specifically, soft biological tissues contain cells in attachment with the matrix. The water content, in most soft tissues, is approximately 55–99% and collagen 0–35%, dependent on location and functional ability. These mechanical properties and structural characteristics of the tissues determine the thermal conductivity and heat transfer during laser irradiation. Water or collagen are the main tissue constituents absorbing the radiation. The thermal denaturation of the collagenous matrix depends on the molecular structures of the collagen fibrils. Older tissues, i.e. in aging, contain more dense collagen fibers and cross‐linking, which provides increased thermal stability and further increased temperature to allow tissue shrinkage and stress relaxation. Collagen is in general a good thermal insulator. Therefore, tissues with more collagen have lower thermal conductivity; therefore, cannot be excised easily with laser.
In general, collagen becomes denatured in a temperature of approximately 60 °C, and this temperature may be higher in older tissues with higher collagen density, where no stress relaxation was observed in temperatures higher than 100 °C for several minutes (Le Lous et al. 1982).
These phenomena of stress relaxation and tissue shrinkage occur faster when the structural integrity in the tissue is less dense or when microfibrillar collagenous structures are present. For instance, the presence of the fibrillar collagen type V, which is predominant in the granulation and inflamed tissues, has an impact to the mechanical instability even after short periods of irradiation.
When longer wavelengths (far‐infrared spectral region) are applied, the water absorption peaks up, and therefore absorption dominates over scattering. In contrast to this effect, the optical absorption properties in pigmented and highly vascularized tissues are predominant in the red and near infrared spectrum, due to the presence of the biomolecules hemoglobin (Hb) and oxyhemoglobin (HbO2) in those tissues.
2.2 Wound Healing and Low Power Lasers
The first studies on low power lasers came from the group of Mester et al. (1969, 1971). They observed a biostimulatory effect on the skin of mice after the use of a ruby laser. The same effect was also achieved in dogs and pigs with a GaAs laser (Cho and Cho 1986; Sapeira et al. 1986). Studies of rat skin showed a raised tensile strength and quicker healing of wounds. The exact mechanism of function is still not yet clear.
Recently, many studies showed that low‐level laser therapy (LLLT) can accelerate the repair of different tissues in the body, leading to inflammatory, immunological, and metabolic effects (Sun and Tunér 2004; Nussbaum et al. 2009; Demir et al. 2010). Low power lasers do not have such effects but act on the tissue thermally to increase the rate of repair of the injured tissue (Yu et al. 1996).
Abergel et al. (1984) researched the effect of the Nd:YAG laser and Kana et al. (1981) of the argon laser on wound healing. In the current literature one finds mostly positive information about the biostimulatory effect of the laser light (Table 2.1) after use of He:Ne lasers (Kovacs et al. 1974; Mester et al. 1974; Sapeira et al. 1986; Abergel et al. 1987; Lyons et al. 1987; Braverman et al. 1989; Hans et al. 1992) or of GaAs lasers (Abergel et al. 1984; Cho and Cho 1986; Anneroth et al. 1988; Asencis‐Arana and Martinez‐Soriano 1988; Takeda 1988a; Braverman et al. 1989). Most studies are in vitro experiments (e.g. with cell cultures, biochemical measurements, like identification of type I and type III pro‐collagen, RNA analysis, collagen synthesis, etc.) or animal studies.
The dental studies of Takeda (1988a) gave the histological proof of a higher fibroblast proliferation and quicker osseous healing after GaAs‐irradiation of fresh extraction sockets of rats. Directly after tooth extraction, the wounds were irradiated for five minutes with a GaAs laser (904 nm and light intensity of 25 mW/cm2). This irradiation was continued the next day. In another study Takeda (1988b) ascertained a raised salivary gland function after use of light with a wavelength of 904 nm.
Trelles and Mayayo (1987) carried out studies about the osseous healing of mice. Fractured bones were irradiated for 21 days with a He:Ne laser (total dose: 2.4 J every other day). The study showed a clear increase of vascularization and osseous density in the fracture region.
After a He:Ne‐irradiation of dentin surfaces at in vivo investigations of monkeys (Matsumoto et al. 1985, 1988) or human wisdom teeth (Kondo et al. 1988), no histological changes of the pulp were observed. Other studies showed a reduction of pain at the orofacial area after use of such lasers (Bezuur et al. 1988), as well as of the cervical tooth sensitivity (Renton‐Harper and Midda 1992).
In the literature, there is a partly controversial discussion about the effect of laser light on wound healing (Table 2.1). The studies of Brunner et al. (1984) could not confirm a positive effect of the krypton laser light on epidermis regeneration. Also Colver and Priestley (1989) could prove no stimulatory effects of the He:Ne on epithelial cells, fibroblasts, and collagen synthesis. Other studies with different tissues showed contradictory effects of the soft lasers on wound healing or a dependence on the applied energy settings and the chosen wavelengths.
Recent studies by Fekrazad et al. (2015) showed the impact of different color lasers (blue, green, and red) on the healing of oral wounds in diabetic rats. The investigators compared the effects of red, green, and blue lasers in terms of accelerating oral wound healing in diabetic rats. The findings suggested that wound healing occurs faster with a red (630 nm) laser compared to blue (425 nm) and green (532 nm) lasers. The energy density was 2 J/cm2 with a treatment schedule of 3 times/week for 10 days. A faster and more organized reepithelialization and tissue healing of the oral mucosa were achieved with an energy density of 4 J∕cm2 in comparison to 20 J∕cm2 (Wagner et al. 2013).
Other studies (Rupel et al. 2018) also showed that various wavelengths differentially modulate reactive oxygen species (ROS) production. In particular, the 660 nm laser light increases ROS production when applied either before or after an oxidative stimulus. The 970 nm laser light exerted a moderate antioxidant activity in the saliva of cancer patients with oral mucositis (OM) who receive chemotherapy or radiotherapy. The most marked reduction in the levels of ROS was detected in cells exposed either to the 800 nm laser light or to the combination of the three wavelengths. Overall, the study demonstrates that PBM exerts different effects on the redox state of both PMNs and keratinocytes depending on the used wavelength and prompts the validation of a multiwavelength protocol in clinical settings.
Table 2.1 Wound healing effects of the low‐power lasers.
Study | Laser type | Tissue | Examined parameters | Results |
---|---|---|---|---|
Mester et al. (1969) | Rubin | skin (mouse) | macroscopy /histology | + |
Mester et al. (1971) | Rubin | skin (mouse) | macroscopy / histology | + |
Kovacs et al. (1974) | He:Ne | skin (rat) | tensile strength | + |
Mester et al. (1974) | Rubin | skin tumors (human) | macroscopy electron microscopy | + |
Hutschenreiter et al. (1980) | He:Ne | skin (rat) | tensile strength macroscopy histomorphometry | no differences |
Haina et al. (1981) | He:Ne | skin (rat) | granulation tissue | + |
Kana et al. (1981) | Ar, He:Ne | skin (rat) | collagen synthesis macroscopy | + |
Abergel et al. (1984) | Nd:YAG, He:Ne, Ar | fibroblast culture | collagen synthesis | + |
Brunner et al. (1984) | Kr | skin (human) | epidermis regeneration | no differences |
Matsumoto et al. (1985) | GaAlAs | dentin (monkey) (monkey) | pulp | no differences |
Cho and Cho (1986) | Ga‐As | oral mucosa (dog) | histology | + |
Sapeira et al. (1986) | He:Ne | skin (pig) | biochemistry | + |
Abergel et al. (1987) | He:Ne | fibroblast culture | collagen synthesis | + |
Lyons et al. (1987) | He:Ne | skin (mouse) | tensile strength collagen synthesis | + |
Trelles and Mayayo (1987) | He:Ne | bone mouse | vascularization bone density | + |
+ | ||||
Anneroth et al. (1988) | Ga‐As | skin (rat) | macroscopy histology | no differences |
Asencis‐Arana and Martinez‐Soriano (1988) | He:Ne | colon (rat) | tensile strength | + |
Kondo et al. (1988) | He:Ne | dentin (human) | pulp histology | no differences |
Matsumoto et al. (1988) | He:Ne | dentin (monkey) pulp | no differences | |
Takeda (1988a) | Ga‐As | bone (rat) | histology | + |
Takeda (1988b) | Ga‐As | salivary gland (rat) | function salivary flow | + |
+ | ||||
Braverman et al. (1989) | Ga‐As, He:Ne | skin (rabbits) | tensile strength histology | no differences |
Colver u. and Priestley (1989) | He:Ne | fibroblast‐culture | collagen synthesis | no differences |
Hans et al. (1992) | He:Ne | fibroblast culture | collagen synthesis | + |
Smith et al. (1992) | He:Ne | skin (pig and rat) | macroscopy | scar tissue formation |
+ positive result.
− delayed wound healing.
In contrast to wound healing in the oral mucosa, systematic reviews were able to evaluate the efficacy of laser therapy to improve alveolar healing after tooth extractions. The current available evidence in the literature showed that laser therapy improved the wound healing process, but these findings were limited to the type of laser applied and its specific settings. The most widespread organized bone formation in the extraction socket was observed in the gallium‐aluminum‐arsenide laser group with the energy dose of 10 J/cm2 (Cirak et al. 2018).
Further well‐designed and randomized controlled trials are needed to support a beneficial effect of using laser therapy after tooth extraction (Lemes et al. 2019). Because there are currently still no reproductive results given, the effects of these lasers on wound healing should be evaluated critically.
2.3 Wound Healing and High‐Power Lasers
Hard lasers or the so‐called “optical scalpels” are applied in oral surgical procedures. Depending on the wavelength, there is a different influence on the wound healing process. A large number of clinical observations exists in the literature, which show the effects of laser irradiation on the tissues using histological, immunohistochemical, and electron‐microscopic methods (Table 2.2).
This part of the present chapter is focused on the typical changes of the tissues during wound healing and an overview about the wound healing processes after laser use.
During laser irradiation, light energy will be absorbed and transformed into heat. Heating of cells and tissues can produce reversible injury that can be repaired by host and cellular mechanisms. When energy is higher, irreversible damage leads to tissue death immediately or after the irradiation.
Incisions have different qualities and are associated with the laser wavelength, the power settings, and the laser‐tissue interactions. The cutting efficiency seems to be better for the CO2 and Er,Cr:YSGG lasers compared to Er:YAG or a 980 nm diode laser with noninitiated tip (Figure 2.1). Temperature increase of the tissue during laser irradiation is always fundamental in order to avoid complications and nonreversible tissue damages. The lowest temperature increase occurs using an Er,Cr:YSGG laser (Figure 2.2).
2.3.1 Wound Healing and CO2 Laser
Three different laser systems (CO2, Nd:YAG, and argon) were used in animals to compare histologically the wound healing of tissues. Only the CO2 laser produced a good incision quality; however, wound healing was delayed for approximately four to five days compared to the scalpel. A delay of wound healing (up to 11 days) was also shown by Luomanen (1987) performing incisions on rat mucosa. The study showed an increased infiltration of the connective tissue and delay of wound healing due to the effect of the lateral heat transfer during the CO2 laser incision (Howard et al. 1997). Another immunohistochemical study of the connective tissue showed remarkable, resistant (extracellular) matrix formation after laser irradiation (Luomanen et al. 1987). The foreign body reaction of the laser‐induced wounds was more distinctive (Filmar et al. 1989). In comparison to the scalpel incision, a delayed wound healing took place on pigs after use of the CO2 laser and the electrosurgery (Arashiro et al. 1996). The tensile strength of these wounds was decreased (Ben‐Baruch et al. 1988; Hambley et al. 1988).
Positive results of wound healing after laser use have been proven by Pogrel et al. (1990), with a distinct acceleration of the epithelialization in comparison to cryosurgical and conventional scalpel incisions. Gaspar et al. (1991) histologically confirmed the excellent hemostatic effect of the CO2 laser as well as the extremely low thermal damage of the tissue in comparison to electrosurgery.
Of great importance is also the removal of pathological alterations with the CO2 laser in esthetically critical regions, because no scarring could be observed (Gaspar and Toth 1990). On the other hand, Kardos and Ferguson (1991) have not observed any clear differences between laser incisions and cryosurgery with regard to the wound healing process. Wound healing after CO2 laser induced incisions was compared to scalpel incisions on beagle dogs (Fisher et al. 1983). The healing of the laser wounds developed without damage to the adjacent tissues. Initially, a coagulum of denatured protein formed on the surface; the inflammatory reaction was less; fewer myofibroblasts were present and there was little wound contraction; less collagen was formed and epithelial regeneration was delayed and more irregular.
Previous studies also from our group showed that healing in oral wounds after incisions in the soft tissue in the palate of monkeys is delayed when a CO2 laser was used. The tissue heals delayed and the healing process is slower when the power settings are relatively high. Specifically, the healing was examined after irradiation of the tissue using a noncontact CO2 laser and 2 W, 4 W, and 6 W continuous wave (CW) power in comparison with the scalpel incision. In general, the study showed that the healing was similar with the conventional scalpel incision but only delayed (Romanos et al. 1999).
Table 2.2 Wound healing effects of the high‐power lasers.
Study | Laser type | Tissue | Examined parameters | Results |
---|---|---|---|---|
Verschueren and Oldhoff (1975) | CO2 | bone | histology | − |
Clayman et al. (1978) | CO2 | bone | histology | − |
Horch and Rehrmann (1978) | CO2, Nd:YAG, Ar | mucosa (rabbit) | cutting effect | + |
− | ||||
Small et al. (1979) | CO2 | bone (rabbit) | histology | − |
Horch and Keiditsch (1980) | CO2 | bone (dog) | histology | − |
Gertzbein et al. (1981) | CO2 | bone | histology | − |
Pao‐Chang et al. (1981) | CO2 | bone | histology | − |
Fisher et al. (1983) | CO2 | mucosa (dog) | histology | + |
Luomanen (1987) | CO2 | tongue (rat) | histology | − |
Luomanen et al. (1987) | CO2 | tongue (rat) | immunohistochemistry | resistance in the matrix |
Ben‐Baruch et al. (1988) | CO2 | skin (pig) | tensile strength | − |
Hambley et al. (1988) | CO2 | skin (pig) | tensile strength histology | − |
Nuss et al. (1988) | Ho:YAG | bone ablation | histology | |
Tawakol et al. (1988) | Nd:YAG | skin (rabbit) | tensile strength | no differences |
Filmar et al. (1989) | CO2 | uterus (rat) | histology | − |
Myers et al. (1989) | Nd:YAG | skin (rat) | cutting effect | + |
Nelson et al. (1989a) | Er:YAG | bone rabbit | histology | − |
Schmelzeisen et al. (1989) | Nd:YAG | mucosa (rabbit) | histology | + |
Walsh and Deutsch (1989) | Er:YAG | bone cartilage | histology | + |
Gaspar and Toth (1990) | CO2 | mucosa (rat) | histology | no scar |
Gonzales et al. (1990) | Er:YAG | bone ablation, cartilage | histology | |
Pogrel et al. (1990) | CO2 | skin (rat) | histology | + |
Stein et al. (1990) | Ho:YAG | bone ablation | histology | |
Dressel et al. (1991) | XeCl | bone (rabbit) | histology | no differences |
Gaspar et al. (1991) | CO2 | mucosa (rat) | histology | + |
Kahle et al. (1991) | Er:YAG | cornea (monkey) | immunohistochemistry | + |
Kardos and Ferguson (1991) | CO2 | tongue (sheep) | histology | no differences |
Keller et al. (1991) | Er:YAG | bone (dog) | histology | + |
White et al. (1991) | Nd:YAG | mucosa (human) | cutting effect | + |
Hendler et al. (1992) | Ho:YAG | TMJ | arthroscopy | + |
Kautzky et al. (1992) | Ho:YAG | tongue (rat) | histology | no differences |
Lustmann et al. (1992) | ArF | bone (rat) | histology | − |
Forrer et al. (1993) | CO2 | bone | histology | − |
Koslin and Martin (1993) | Ho:YAG | TMJ | arthroscopy | + |
Romano et al. (1994) | Ho:YAG, Er:YAG | bone | histology | better with Er:YAG |
Moritz et al. (1995) | Er:YAG | TMJ ablation | histology | |
Romanos et al. (1995a, b, c) | Nd:YAG | skin (rat) | histology macroscopy Immunohistochemistry | similar to scalpel scar stable matrix |
Arashiro et al. (1996) | CO2 | skin (pig) | histology | − |
Howard et al. (1997) | CO2 | skin (rat) | histology | − |
Romanos et al. (1999) | CO2 | palate (monkey) | histology | delayed healing compared to scalpel |
+ accelerated wound healing.
− delayed wound healing.

Figure 2.1 Incision quality during laser irradiation of the bovine mucosa showing different penetration depths and carbonization zones. The best incisions occurred using an Er,Cr:YSGG or CO2 laser. The diode laser incision was performed without initiated tip.
Source: Dr. Georgios E Romanos.

Figure 2.2 Temperature changes of the tissue during laser irradiation. The lowest temperature increase occurs using an Er,Cr:YSGG laser (unpublished data provided from incisions in the bovine tongue).
Incisions created by the scalpel, electrocautery, CO2 laser, and potassium titanyl phosphate (KTP) laser in rat tongues were evaluated in terms of wound healing (Carew et al. 1998). The study showed that oral intake, indirectly assessed by postoperative weight loss, by the third postoperative day was significantly decreased in the electrocautery, CO2 laser, and KTP laser groups as compared with the scalpel group. The depth of wound healing, as assessed by histologic examination, was successively greater for the scalpel (75 ± 13 μm), electrocautery (110 ± 10 μm), CO2 laser (145 ± 10 μm), and KTP laser (195 ± 23 μm) groups. Wounds created by the KTP laser had the lowest strength (76.5 ± 6.9 kPa) as compared with the CO2 laser (156 ± 28.4 kPa), electrocautery (153 ± 15.7 kPa), and scalpel groups (249 ± 61.8 kPa).
Also, clinical studies were conducted in order to compare clinical and histopathological outcomes for excisional biopsies when using pulsed CO2 laser versus Er,Cr:YSGG laser. Patients with a fibrous hyperplasia in the buccal mucosa were randomly allocated to the CO2 or the Er:YAG laser group. Intraoperative bleeding occurred in 100% of the excisions with Er:YAG and 56% with CO2 laser. The median thermal damage zone was 74.9 μm for CO2 and 34.0 μm for Er:YAG laser. It was concluded that for excision of oral soft tissue lesions, CO2 and Er:YAG lasers are both valuable tools with a short time of intervention and postoperative low pain. More postoperative bleeding occurs with the Er:YAG than CO2 laser, but the lower thermal effect of the Er:YAG laser seems advantageous for histopathological evaluation (Suter et al. 2017).
A previous study investigated and compared the clinical and histopathologic characteristics of CW and pulsed CO2 laser modes for excisional biopsies. No statistically significant differences in the duration of the laser excision were found between laser modes. The width of the thermal damage zone (micrometers) was expected to be smaller for the pulsed CO2 laser mode (CF group), but there was no statistically significant difference in the widths of thermal damage zones between the CW and CF groups (Suter et al. 2012).
In contrast to this study, other authors presented that heat, sufficient to damage tissue, was conducted to adjacent tissue during laser pulses of 100 μs and longer, like in the conventional surgical CO2 lasers for soft tissue excisions. However, surgical CO2 lasers with short pulses of approximately 60 μs or less could offer more prompt wound healing, while maintaining the advantages of a 10 600 nm wavelength (Fortune et al. 1998).
Similarly, Sanders and Reinisch (2000) compared the thermal damage and wound healing of a 7.5 μs‐pulsed CO2 laser with scalpel and CW CO2 laser incisions. Specifically, incisions on the dorsal pelts of rats with a 7.5 μs pulsed CO2 laser at a 5‐, 10‐, or 15‐Hz repetition rate were compared with a conventional CW laser, or scalpel. Using a 7.5‐μs pulse duration, CO2 laser incisions healed at a rate similar to scalpel incisions and reduced the wound healing process, which was delayed with typical surgical CO2 lasers.
Therefore, CO2 lasers and tissue healing are associated with the application mode and the manufacturer device features, which may be fundamental in order to control heat transfer to the tissues and lateral coagulation zone in order to accelerate the wound healing process.
2.3.2 Wound Healing and the Nd:YAG Laser
Various studies were also carried out to clarify the effect of the wavelength of the Nd:YAG laser on wound healing (Table 2.2). Schmelzeisen et al. (1989) have observed on rabbits after laser incisions and three weeks of healing a complete reepithelialization in comparison to cryosurgical incisions. Wound healing was clearly accelerated, while the tensile strength was low.
With the use of a pulsed Nd:YAG laser, a strong coagulation took place in the area of the incision. A minimal injured zone was histologically proved on rats. White et al. (1991) also showed a low thermal damage in the incision borders on the oral mucosa.
Studies confirmed the positive effect of the laser use by animal‐experimental studies on the skin of rats. Scarring was not present in comparison to the conventional scalpel incisions. Wound healing after the use of a Nd:YAG laser, set at low power, appeared not only clinically and histologically (Romanos et al. 1995a, b) but also immunohistochemically (Romanos et al. 1995a, c) to succeed, and similarly to the scalpel incision (Figures 2.3–2.5) and the extracellular matrix of the connective tissue, remains imperishable and stable (Romanos et al. 1991). If the power of the beam is high, wound healing is delayed, and it can be compared to wound healing after electrosurgery (Romanos 1997) (Figures 2.3–2.5). Synoptically, the results of the wound healing that were observed on rat skin four weeks after Nd:YAG laser use are shown in the Table 2.3.
Full thickness incisions were made in rat skin using a diode laser (805 nm, 10 W, contact mode), a Nd:YAG laser (1064 nm, 10 W, contact mode), and a stainless steel scalpel blade (control). Wound breaking strength measurements were obtained at 7, 14, and 21 days using a specially designed tensiometer. The study showed that there was no significant difference in the breaking strengths (group 1) or tensile strengths (groups 2 and 3) of the diode and Nd:YAG laser incisions. As predicted, breaking strengths and tensile strengths of scalpel blade incisions were significantly greater than those of incisions made with laser energy. Histopathologic evaluation revealed that through day 14, the degree of inflammation and collagen production was similar for diode and Nd:YAG laser incisions. Laser incisions had greater inflammation and a lag in fibroblast invasion and collagen production compared with scalpel incisions. By day 21, all incisions were similar in fibroblast population and collagen production, but laser incisions had slightly more inflammation than scalpel incisions. The authors concluded that in the primary wound healing model described, the tissue effect, cellular response, and development of wound strength were essentially the same for the high‐power diode laser at 10 W and the Nd:YAG laser at 10 W (Taylor et al. 1997).

Figure 2.3 Wound healing after scalpel incision (a); 1.75 W power Nd:YAG (b); 3 W power Nd:YAG (c); or after electrosurgery (d); four days after surgery.
Source: Dr. Georgios E Romanos.

Figure 2.4 Wound healing after scalpel incision (a); 1.75 W power Nd:YAG (b); 3 W power Nd:YAG (c); or after electrosurgery (d); two weeks after surgery.
Source: Dr. Georgios E Romanos.

Figure 2.5 Wound healing after scalpel incision (a); 1.75 W power Nd:YAG (b); 3 W power Nd:YAG (c); or after electrosurgery (d); four weeks after surgery. The low power Nd:YAG laser wound does not demonstrate any signs of tissue discoloration (b) compared to the scalpel incision (a).
Source: Dr. Georgios E Romanos.
Table 2.3 Wound healing in the rat skin, four weeks after Nd:YAG laser irradiation.
low power (1.75W) Nd:YAG Laser | scalpel | electrosurgery | high power (3W) Nd:YAG Laser | |
---|---|---|---|---|
pigmentation | − | + | + | + |
scar | − | − | + | + |
wound healing process delayed | ++ | ++ | + | + |
granulation tissue | +++ | +++ | ++ | + |
formation | ||||
stable and collagen | +++ | ++ | + | + |
resistant matrix | ||||
necrosis | + | ++ | ++ | +++ |
A recent study aimed to investigate the capacity of bone repair using a high‐power, Q‐switched, pulsed, Nd:YAG laser, using bilateral calvarial defect animal models, with noncritical sized 5 mm (rat) or 8 mm (rabbit) diameter (Kim et al. 2015). Laser irradiation significantly increased new bone formation by approximately 45%, not only in the sponge‐filled defects of rats but also when the defects were left empty, compared to the nonirradiated group. Consistently, both doses of output power (0.75 and 3 W) enhanced new bone formation, but there was no significant difference between the two doses. This study is one of the first to demonstrate the beneficial effect of Nd:YAG lasers on the regeneration of bone defects which were left empty or filled with collagen sponge, suggesting its great potential in postoperative treatment targeting local bone healing.
The fact that there are various results described in the literature for the use of laser is presumably caused by several factors, e.g. the wavelength and power setting used in each case, as well as the kind of the irradiated tissue and species. Therefore, a critical judgment of the results by the active clinician is of utmost importance.
2.3.3 Wound Healing and Other Laser Wavelengths
Wound healing studies evaluate the use of different laser wavelengths with specific power settings and application parameters in comparison with other conventional methods or application of different incisions.
Previous studies performed in monkeys’ corneas after the use of Er:YAG and excimer lasers (ArF). The healing with the excimer laser appeared to be worse, compared to the Er:YAG laser, although with this laser type the thermal damage was lower (Kahle et al. 1991). Other studies with excimer (XeCl, 308 nm) and pulsed Ho:YAG laser were carried out on the tongue mucosa of rats. The Ho:YAG laser showed an impressive hemostasis. The wound healing process was similar, even though the temperature of the irradiated tissue during the use of the Ho:YAG laser was higher (Kautzky et al. 1992).
Unpublished data from our group showed that, in palatal incisions in monkeys, the impact of a diode 980 nm laser is similar to scalpel wound healing, when noninitiated tips are used in contact with the oral mucosa. Specifically, a 5‐W diode laser demonstrates a complete wound closure after three days with reepithelialization, formation of long rete pegs, and complete organization of the epithelial layer (Figure 2.6, unpublished data).
In contrast, a wound healing delay was found when the power was higher, such as 10 W, demonstrating the closure of the wound with a pseudo‐membranous epithelial coverage (Figure 2.7, unpublished data). High power of 15 W was associated with high temperature changes and showed a delayed wound healing after two weeks but without tissue necrosis. Specifically, exudation, microhemorrhage, and blistering (vacuole formation) due to the high heat transfer were observed in palatal wounds (Figure 2.8, unpublished data).
Compared to the traditional scalpel, the incision of the Er:YAG laser provides a smaller inflammatory reaction, more pseudomembranous formation, and minimal damage of the tissue in palatal mucosa of rats (Qu et al. 2018).
In contrast to the previous laser systems, recent studies evaluated the 445 nm semiconductor laser (blue light) for tissue incision and demonstrated an effective cut as expected due to the special absorption properties of blue laser light in soft tissues. Forty soft tissue specimens were obtained from pork oral mucosa and mounted on a motorized linear translation stage. The handpiece of a high‐frequency surgery device, a 970 nm semiconductor laser (with initiated fiber, contact mode at 3 W CW), a 445 nm semiconductor laser with noninitiated fiber tip, and a high‐frequency surgery device with straight working tip were used to make incisions.

Figure 2.6 Incision area three days after 5W diode laser (980 nm) incision presenting complete wound closure with normal epithelium layers and long rete pegs.
Source: Dr. Georgios E Romanos.

Figure 2.7 Incision area 14 days after 10W diode laser (980 nm) presenting complete wound closure with pseudo‐membrane on the epithelial surface. This provides evidence about the delayed wound healing using this power setting.
Source: Dr. Georgios E Romanos.

Figure 2.8 Incision area 14 days after 15W diode (980 nm) laser presenting microhemorrhage and blistering due to the high heat transfer to the tissue especially in the subepithelial layer.
Source: Dr. Georgios E Romanos.
The highest incision depth was achieved with the 445 nm laser contact mode demonstrating the lowest amount of soft tissue denaturation. The lowest incision depth was measured for the high‐frequency surgical device. Using a 445 nm semiconductor laser, a higher cutting efficiency can be expected when compared with a 970 nm diode laser and high‐frequency surgery. Even the 445 nm laser application in noncontact mode shows clinically acceptable incision depths without signs of extensive soft tissue denaturation (Braun et al. 2018).
A recent report compared the efficacy and precision of femtosecond laser ablation with conventional surgical laser devices. Femtosecond lasers do not provide collateral thermal damage and therefore there are increased applications in number and diversity of these lasers. Future innovations in laser design will make them more accessible, facilitating clinical and research applications (Chung and Mazur 2009).
2.4 Lasers and Bone Healing
Osteotomies with lasers were carried out for the first time during the 1970s (Goldman et al. 1970); however, such procedures were not routinely applicable until recently in private practice (see Chapter 4). Collateral thermal damage and residual char formation have severely limited the use of conventional lasers in the surgical preparation of bony tissue. This thermal damage from lasers can be controlled using specific laser wavelengths that are strongly absorbed by the bone and by reducing laser pulse durations.
Morphologic and chemical changes were induced in the near‐surface region of bone following exposure to the free electron laser (FEL) at 3.0, 6.1, and 6.45 μm wavelengths. The selected wavelengths coincide with the vibrational modes of proteins and water within bone. Laser parameters included 22.5 ± 2.5 mJ/pulse delivered in individual 4 μs macropulses at a repetition rate of 30 Hz, focused to 200 and 500 μm spot sizes.
The light microscopy sections of the ablation defects created at the differing wavelengths showed similar features, i.e. two zones of collateral damage: a zone generally <10 mm of extensive thermal damage and a wider zone of empty lacunae (Spencer et al. 1999). The spectroscopic results suggested that the char layer is limited to an area less than approximately 6 μm from the surface.
Thermal damage and two to three weeks delay of wound healing were presented earlier (Schmelzeisen et al. 1989). Studies with the pulsed excimer laser on the shinbone of rats (Lustmann et al. 1992) and with the Er:YAG laser on rabbits (Nelson et al. 1989a, b) led to similar results. Most studies of laser‐induced CO2 osteotomies showed a delayed wound healing because of the increased temperature increase leading to thermal damage of the bone (Verschueren and Oldhoff 1975; Clayman et al. 1978; Gertzbein et al. 1981; Pao‐Chang et al. 1981; Forrer et al. 1993). Forrer et al. (1993) confirmed with their studies that the pulse rate and the energy setting are closely related to an osseous necrosis and, hence, short pulse duration is recommended to obtain controlled tissue damage.
In contrast, no differences in the osseous healing could be ascertained after the osteotomy with an excimer (XeCl) laser or with custom surgical bone‐cutting burs (Dressel et al. 1991).
Comparative studies evaluated the healing of bone in rat tibial osteotomy defects created by a dental bur, a CO2 laser with and without removal of the char layer, and a Nd:YAG laser with char layer removed and with and without use of an air/water surface cooling‐spray (Friesen et al. 1999). Tibial osteotomy defects were created in four groups of rats each using the following: (i) #6 round bur with simultaneous saline irrigation; (ii) CO2 laser with char layer intact; (iii) CO2 laser with char layer removed; (iv) Nd:YAG laser with air/water surface cooling and char layer intact; (v) Nd:YAG laser with air/water surface cooling and char layer removed; and (vi) Nd:YAG laser without air/water surface cooling and char layer removed. Both laser types were used at energy densities typically utilized for oral soft tissue surgery. Laser‐induced osteotomy defects, when compared to those prepared by rotary bur, exhibited a delayed healing response that appeared to be related to the presence of residual char in the osseous defect.
Also, other studies compared the effect of CO2 and Nd:YAG laser on the bone. Specifically, the studies were focused on the long‐term effects of laser irradiation after 21, 35, and 63 days posttreatment: (i) a negative control (no treatment); (ii) a positive control (bur osteotomy); (iii) a CO2 laser at 5 W (860 J/cm2); (iv) a CO2 laser at 6 W (1,032 J/cm2); (v) a Nd:YAG laser at 5 W (714 J/cm2); and (vi) a Nd:YAG laser at 7 W (1,000 J/cm2). All laser irradiation was delivered in the presence of a surface coolant consisting of air and sterile water. Under the conditions of this study, the healing was severely delayed in all laser‐treated sites compared to positive control sites. Of the laser‐treated sites, those irradiated by the CO2 laser at 5 W exhibited the greater amount of bone regeneration. In general, the osseous healing response was severely delayed by CO2 and Nd:YAG laser irradiation of bone, even in the presence of a surface cooling spray of air/water (McDavid et al. 2001).
The healing of beagle dogs was also the same after the use of a Er:YAG laser (Keller et al. 1991). The Er:YAG laser allows ablation of bone, as well as cartilage (Tawakol et al. 1988; Nelson et al. 1989a; Gonzales et al. 1990) and, therefore, is used today, at least experimentally, in the surgery of the temporomandibular joint (Moritz et al. 1995). The thermal damage of an Er:YAG laser–osteotomized bone is lower than with the Ho:YAG laser (Romano et al. 1994). Currently, only the Er:YAG laser is the most suitable in orthopedics and experimentally qualified in arthroscopy.
Bone healing in an inferior border defect of the rat mandible was examined using either an Er:YAG laser or a mechanical bur for drilling (Lewandrowski et al. 1996). The histologic evaluation demonstrated no difference in the amount of newly formed woven bone at the osteotomy site or screw holes made by either the Er:YAG laser or the drill. The extent of thermal damage at the osteotomy sites was comparable in laser and mechanically cut bone fragments. Based on this study, the Er:YAG laser can be used clinically in thin, fragile bones in the maxillofacial region.
De Oliveira et al. (2016) showed in recent studies the impact of the application of Er:YAG and Er,Cr:YSGG lasers during osteotomy on bovine bone blocks. A conventional bur was compared with an Er:YAG laser and Er,Cr:YSGG laser. Even though the bur was the instrument that performed the osteotomy in the shortest amount of time, all instruments caused thermal damage; however, the Er,Cr:YSGG laser was the only type that induced carbonization, and the Er:YAG laser induced the lowest degree of thermal damage in bone tissue after osteotomy.

VIDEdental - Online dental courses
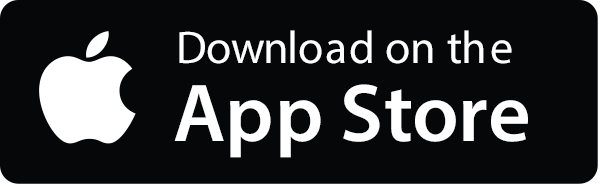
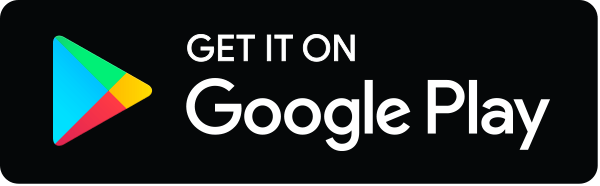