1
Laser Fundamental Principles
Georgios E. Romanos
Stony Brook University, School of Dental Medicine, Stony Brook, NY, USA
LASER is an acronym of “Light Amplification by Stimulated Emission of Radiation.” Laser is light with specific properties and may interact with tissues and materials. Light is an electromagnetic wave, which is a coupling of electric and magnetic fields, traveling as waves at a speed equal to the known speed of light (velocity, c). Both fields oscillate at the same frequency, with a number of oscillations per second, which is well known as frequency ( f ). The speed of light is a universal constant, which is about 300 000 km/s.
Since medical professionals are interested in the applications of laser devices and not the internal physics, here we describe fundamental information, which is foundation knowledge, before the use of lasers in clinical settings.
A laser light is a monochromatic, coherent light in the visible and nonvisible (infrared or ultraviolet [UV]) parts on the electromagnetic spectrum. Laser light is optical radiation and is termed non‐ionizing radiation to be differentiated from ionizing radiation, such as gamma‐ and X‐rays, which may cause biological effects in the cells and tissues. The human eye associates a color to a group of specific wavelengths from violet, blue, green, yellow, orange, red based on the increase of the wavelengths. Invisible wavelengths for the human eye are wavelengths of radios and television (infrared) or in the UV parts of the spectrum, the gamma‐ and x‐rays (Figure 1.1).
The spectrum is divided into two major zones: the short wavelength ionizing radiation (nonvisible to the human eye) and the non‐ionizing radiation (visible light and nonvisible infrared radiation) with longer wavelengths. The ionizing radiation can penetrate tissue and damage cells. In low doses it can be used for diagnostic purposes (i.e. X‐rays). The non‐ionizing range of radiation can be used for superficial heating of tissues, and for treatment of skin disorders and musculoskeletal injuries.
The power of lasers can range from milliwatts to almost 20 W for commercial lasers. In addition, higher levels of power in megawatts may be used for military purposes.
The sizes of lasers can have dimensions larger than 100 m. Lasers in this size can be used for nuclear experiments using laser beams to squeeze hydrogen atoms in order to release a high amount of energy (laser fusion). The biggest facilities in the world so far are the NIF (National Ignition Facility) in California and the Laser Megajoule (LMJ) in France, near Bordeaux.
In contrast to large lasers, the smallest lasers today are 5000 times smaller than the tip of a pen. Scientists have created the world’s smallest laser after they squeezed light into a space smaller than a protein molecule. The so‐called “spacer” generates stimulated emission of surface plasmons (oscillations of free electrons in metallic nanostructures) in resonating metallic nanostructures adjacent to an active medium. It is anticipated that, at least experimentally, the spacer (wavelength of 531 nm) will advance our fundamental understanding of nano‐plasmonics and the development of new opportunities due to the photothermal properties in the therapy of malignant lesions (Chon et al. 2014).
In general, there is a broad diversity in laser applications, which can be used for industrial, commercial, research, and military interests.
Some areas where lasers can be used are:
- Material cutting and welding
- Measurements
- Communications
- Entertaining and performing arts
- Holography
- Spectroscopy and atomic physics
- Environment protection
- Plasma diagnostics
- Medical applications

Figure 1.1 Electromagnetic spectrum and the different wavelengths.
There is no way to think about modern life without the internet, mobile phones, and technology. Therefore, lasers are everywhere in our lives since lasers are fundamental in all these technological advances.
Lasers can do a lot, for example measuring distances, such as the depth of oceans and in aerospace, based on the principle that laser light is sent to a target, which will then be reflected and sent backward. For instance, laser light can be sent to the moon, collecting a few photons reflected back by mirrors placed on the lunar surface (such as during the Apollo missions), and then we know the distance between the moon and the Earth.
The coherent properties of laser light will be used in ring laser gyroscopes allowing distance measurement in aircrafts, helicopters, missiles, ships, etc. Bar code readers and scanners exist only in conjunction with diode lasers. Also, optical storage capacity from compact discs (CDs) to digital video discs (DVDs) and today Blu‐ray discs depends on the density of coding elements (pits) and the laser spot after focusing. The shorter the wavelength, the smaller the laser spot and the engraved surface of the disc. In addition, partial or complete absorption of the light can be at resonance with the material medium and create distinguished resonance frequencies (signals), characterizing the medium composition (spectroscopy).
In medicine, cornea surgery, removal of wrinkles, and coagulation of blood vessels in abdominal surgery accommodate lasers in daily practice. Also, other applications in laser medical imaging, like the phenomena of scattering and absorption of light by tissues, have been used extensively the last few years establishing excellent opportunities in the field of diagnostics. Specifically, optical coherence tomography (OCT) today allows a high‐resolution cross‐sectional imaging compared to the conventional diagnostics due to the reflected light by a mirror and by measuring backscattered or back‐reflected light.

Figure 1.2 OCT device for clinical and diagnostic applications.
Source: Dr. Georgios E Romanos.
OCT (Figure 1.2) can provide cross‐sectional images of tissue structure on the micron scale in situ and in real time. This relatively new technology is very helpful today in biomedical and clinical sciences. Especially in ophthalmology, it provides treatment guidance for glaucoma and diseases of the retina, including age‐related macular degeneration (AMD) and diabetic eye disease (Fujimoto et al. 2000).
1.1 Historical Background
The precursor of the laser, namely the “Maser,” was developed in the United States by the physicist Theodore H. Maiman (1960). It consisted of an one‐crystal‐rod from artificial ruby and could emit red light with a wavelength of 694 nm in the microwave band. The Maser, an acronym for Microwave Amplification by Stimulated Emission of Radiation, is today generally known under the name laser. In its name is summarized the basic principle after which all laser systems work. Charles H. Townes (1964) received the Nobel Prize for the development of the laser; Townes was the first to achieve, due to stimulated emission, the fortification of the radiation in the microwave band.
Moreover, Albert Einstein (1917) had already argued in his thesis “Quantum Theory of Radiation,” that parts of the electromagnetic field can be stimulated in such a way that through it fortified light originates. The first lasers were called optical masers.
1.2 Energy Levels and Stimulated Emission
Based on Niels Bohr and the Planck‐quantum hypothesis, the following two postulates were formulated:
- Electrons move only on certain, firm orbits around the nuclear core
Figure 1.3 Spontaneous and stimulated emission principles.
- Electrons can jump only from orbit to orbit and deliver energy in the form of radiation, as for example light (emission of radiation), or take up energy (absorption of radiation).
Therefore, in the interaction between light and matter three different optical concepts may occur: absorption, spontaneous emission, and stimulated emission.
Absorption is the process when electrons transfer from a low energy level (E1) (stable) to a higher energy level (E1) (unstable). Energy levels E1 are called the ground state and E2 called the excited state.
Spontaneous emission is the process, when electrons transit from a higher energy level (E2) to a lower energy level (E1). When E2 > E1, the energy difference satisfies the relation E2‐E1 = h ν. The constant h (= 6.63 × 10−34 J/s) is known as Planck’s constant, and ν is the radiation frequency. Spontaneous emission is responsible for the production of conventional visible sunlight.
Stimulated emission is the process when atoms initially from the excited stage fall down to the ground state emitting photons. An atom can be stimulated (excited stage) by an external source, so that its electrons of a low energy can jump to a higher energy orbit. This source can be of an electric kind, e.g. a flashbulb, and serves as “a pumping mechanism.” Other pumping methods can be also chemical or optical, depending on the energy source (Figure 1.3).
1.3 Properties of the Laser Light
With the term laser is identified a physical principle leading to the production of electromagnetic radiation, which differs from the usual light in the following properties (Figure 1.4):
- Coherence: Wave streaks remain parallel and well‐defined even in large distances. The light has spatially the same phase (the waves are “in tune”).
- Collimation: The laser beam can give a localized spot when something is in its way. This has the practical advantage that the light can be well focused.
- Monochromatism: All wave streaks have the same wavelength, the same frequency, and thus the same energy. The wavelength of the light plays a critical role in medicine and determines today the exact clinical ranges of application.
A high energy density is produced when the generated electromagnetic radiation bundles in the narrowest space, due to the coherence and the collimation. The light can be focused precisely and have, because of its high energy density, different effects on the tissues. Therefore, vaporization, coagulation, and also carbonization of tissues are possible. Light with such qualities does not exist in nature. The photons of usual light exhibit different wavelengths, and they are emitted in all directions (Figure 1.4) of space (polychromatic, incoherent light).

Figure 1.4 Collimated light of the laser versus non‐collimated light of the conventional light source
The concurrent combination from the above‐mentioned physical properties permits very high capacity density. In this way, for example, the sunlight striking our earth has power of on an average 0.1 W/cm2; on the contrary, surgical laser systems easily reach a power of 100 000 W/cm2. Lighting a match produces energy of 200 J. With the energy of only 1 J of coherent light generated by a ruby laser – focused by means of a plane optical lens – it is possible to cut a hole in a metal plate (Frank 1989).
The three basic criteria of light are: brightness (amplitude), color (frequency), and polarization (angle of vibration).
1.4 The Laser Cavity
From the practical standpoint, a laser device (Figure 1.5) contains the following components:
- The laser medium (active medium), which generates the laser light (this is the “brain” of the system).
- The optical resonator (reflecting system)
- The laser pumping mechanism
1.4.1 Active Medium
Atoms are stimulated to the production of the laser radiation. These atoms are components of the so‐called “active (gain) medium.” This can be a gas, a solid body (crystal), a liquid, or a semiconductor. Different lasers systems can be classified based on the active medium.

Figure 1.5 Schematic demonstration of a laser device.
1.4.2 Pumping Mechanism
The laser pumping mechanism is the act of energy transfer from an external source into the active medium of the laser. The pump energy is usually provided in the form of light (optic) energy, or electrical current, but also other sources have been used, such as chemical or nuclear reactions.
1.4.3 Lenses – Resonator
The optical resonator is the reflecting system of the laser device. With the use of two parallel, arranged mirrors (mostly concave shaped) at a specific distance, the light will be reflected. The exact radius of curvature characterizes the optical resonator. A certain curvature controls better the light reflections, modifying the distribution of light within the laser output beam.
The resonators with a stable reflecting distance are also called also stable resonators and differentiate themselves from the unstable ones, which obtain a variable reflecting distance. According to the distance and shape of the mirrors as well as their position, there are concentric, confocal, hemi‐confocal, and hemispherical resonators. Energy loss can happen if mirrors (especially output mirrors) do not perfectly reflect light, and this should happen as much as possible. Concave mirrors are needed in order to focus light transversely.
The simplest laser cavity is formed by two parallel mirrors facing each other. This is called a Fabry‐Perot Cavity.
The laser resonator has two different types of modes: transverse and longitudinal. Transverse modes can be explained by the cross section of the beam profile and represents the intensity pattern. This distribution of power is also referred to as transverse electromagnetic mode (TEM).
1.5 Laser Application Modes
The operation mode of a laser can be switched to pulsed or continuous (Figure 1.6). The pulsed mode is also known as normal mode. A continuous beam is referred to as continuous wave (“continuous‐wave laser”) or CW laser, when light will be constantly emitted over an uninterrupted period of time due to continuous pumping. These lasers have usually low peak energy and low power. They are usually gas lasers, i.e. CO2 lasers.
The type of the operating mode, namely the length or width of a pulse is dependent on the pumping mechanism and the laser medium. The pulsed laser light (gated, chopped) can be achieved when a mechanical shutter opens and closes in front of the beam.
Pulses can be short or ultrashort dependent on the pulse duration. A superpulse mode is associated with good ablation and wide residual thermal damage (RTD) compared to the ultrapulse mode, where the ablation is precise and the RTD is shallow. The latter may be also called char‐free mode.
Usually pulses have a pulse duration in the μs‐ms range. Free‐running ( FR ) lasers are pulsed lasers with shorter pulse durations than the conventional pulsed lasers. Such lasers can be used in areas when risk of overheating has to be avoided. For instance, a FR‐Nd:YAG is used for the LANAP protocol in periodontal therapy (see also Chapter 5).
Shorter pulses with pulse duration from microseconds (10−6) to nanoseconds (10−9) define the Q‐switched lasers (Q‐switching). Compression or shortening of pulses can be done with this technique. This kind of laser can be used in industry for metal drilling, cutting, and marking with extremely high peak power.
The second compression technique of pulses is to create pulses with extremely short duration; sometimes referred to as ultrashort pulses. These are pulses with a width in picosecond (10−12 seconds), femtosecond (10−15 seconds), or attosecond (10−18 seconds) defining the mode‐locking. This can be used for cutting or melting of metals due to the high penetration depth. Pulse repetition rate (frequency) also varies widely.
Pulse modes control the heat transfer to the tissues, providing vaporization without overheating and, as a consequence, melting. High peak power pulses can create defects with sharp edges in the matter (or tissues) without damage.
There is great interest in the pulse duration, also called pulse width, of the laser beam in order to avoid negative effects and damage in biological tissues.
Chopped (shuttered) pulses usually have a duration of 100–500 ms. Superpulses have a shorter width, usually of 60–200 μs and higher peak power. The width can be controlled electrically using mechanical shutters and other devices, like shutters and Q‐switches. These devices are placed in the laser cavity.
The pulse width must be shorter or equal than the thermal relaxation time ( TRT ) of the target chromophore. This time is directly proportional to the square size of the chromophore. Therefore, small objects cool faster than large ones, while larger chromophores have a longer TRT than smaller chromophores.

Figure 1.6 Continuous (CW) and pulsed (chopped, gated) laser application modes compared to pulsed, superpulse, and ultrapulse mode.
The TRT is defined as the time needed for the target chromophore to dissipate 63% of its peak temperature. Bogdan Allemann and Kaufman (2011) showed different TRTs of importance based on the chromophore size in dermatology (see Table 1.1).
Contact and non‐contact laser modes can be defined dependent on the position of the optic fiber or tip in relation to the tissue or material.
Important parameters, when continuous lasers are used, are the irradiation period, power, and spot size. In contrast, for pulsed lasers maximum energy per pulse, pulse duration, frequency, and spot size are fundamental. Power (in watts) is defined by the transmitted energy (in joules) per unit time.
Therefore,

Also:
- meanP = Pmax × tpulse × frequency
- Pmax is the maximum power (watt)
- tpulse is pulse duration (second)
- frequency (Hz)
Table 1.1 Thermal relaxation times for different chromophores of various size.
Size, μm | Thermal relaxation times (approx.) | |
---|---|---|
Tattoo ink particle | 0.5–4 | 10 ns |
Melanosome | 0.5–1 | 1 μs |
Erythrocyte | 7 | 2 μs |
Blood vessel | 50 | 1 ms |
Blood vessel | 100 | 5 ms |
Blood vessel | 200 | 20 ms |
Hair follicle | 200 | 10–100 ms |
The energy per area is the power density (PD, or fluence) and expressed in joules/cm2
- PD = meanP/S
- PD is Power Density (watt/cm2); mean power (watt); S is the irradiated surface (cm2)
- r is the radius of the glass fiber; (S = π . r2), π = 3.14
All parameters that must be included in different laser studies should be: power density, energy of the laser beam, pulse width and frequency, irradiation period, diameter of the glass fiber (or tip), beam profile, distance to the irradiation object, and tip angulation.
Peak Power is the energy flow in every pulse

Average Power is the energy flow over one full time period.

Therefore:

Also, Duty Cycle is the fractional amount of time the laser is “on” during a specific given period.
Therefore:

1.5.1 Beam Profiles
The energy distribution across the beam (transverse electromagnetic mode) determines the nature of laser focus (focal spot size). This focus can have a circular, clean pattern (TEM00), or an irregular pattern (multimode, TEMxx). The circular spot is the fundamental mode, with Gaussian (normal distribution) in the beam profile, which has the highest concentration of power and can be focused into the smallest, most concentrated focal spot (Figure 1.7). The TEM00 mode is the most desirable beam. The fundamental mode with the maximum intensity peak at the center of the beam is the TEM00 and contains roughly 86% of the power in the spot.
Longitudinal modes correspond to different resonances along the length of the laser cavity which occur at different frequencies or wavelengths. The transverse modes are classified according to the number of nulls that appear across the beam cross‐section. However, multimode beams can have high power but lower quality.

Figure 1.7 Transverse electromagnetic modes with regular, high concentrated beam (TEM00) and irregular (TEMxx) pattern with less concentration of the maximum energy in the beam.
1.6 Delivery Systems
The laser beam is used as a handpiece by means of different guide systems (the so‐called beam guide systems), allowing the surgeon to perform a perfect, with minimal complications, and practical, laser application. A direct coupling, an articulated arm, a flexible hollow guide as an optical fiber, or a fiber system are currently available for this purpose.
1.6.1 Direct Coupling
A direct coupling is possible only in extremely compact systems (e.g. He:Ne target lasers, soft lasers, laser pointers). In such systems the laser unit corresponds to the handpiece of the system.
1.6.2 Articulated Arms
The laser beam can be used as a handpiece by means of articulated arms (Figure 1.8) at specific wavelengths (e.g. in the UV range and the wavelength of the CO2 laser). In such systems, mirrors are used for beam deflection. For this reason, such articulated arms are also called transmission arms.
Articulated arm beam delivery dates back to the 1970s; it features a cumbersome four‐elbow, seven‐mirror articulation, which can rotate to different angles for the transmission of the laser beam, but it can have limitations in accessibility. A lens in the base of the handpiece focuses the laser beam 2–3 cm from the exit aperture of the handpiece. An articulated arm is unusable without an aiming beam – the only visible indicator of the focused CO2 laser beam location on the target tissue.
Although, the beam quality can be described as a very good one, disadvantages are the large weight of the articulated arm and thus the entire laser unit, the relatively inflexible operation, and the relatively expensive reconstruction. To reduce the heavy weight, a technical modification is needed. A weight balance with gas pressure springs or counterweights can also positively affect the clinical use of endoscopes, surgical microscopes, or handpieces.

Figure 1.8 Articulated arm for a CO2 laser application in the modern CO2 laser (Denta 2, Lutronic, GPT dental, Fairfield, NE, USA).
1.6.3 Fiber Systems and Flexible Hollow Guides
These fiber systems are flexible light guides made of glass fibers (high‐purity fused quartz glass) coated with transparent plastic or piping systems, the so‐called waveguides (Figure 1.9).
Flexible hollow guides are also used in the construction of CO2 lasers, instead of more expensive and relatively inflexible articulated arms. The flexible hollow fiber for CO2 laser wavelength was developed in the 1990s; it features an unprecedented reach and accessibility unattainable with articulated arm lasers. A pen‐size, scalpel‐like handpiece is held very close to the target tissue. It focuses the CO2 laser beam 1–3 mm away from handpiece’s distal end; no aiming of the beam is needed. The handpiece is autoclavable, and the latest designs use no disposable tips.

Figure 1.9 Hollow guide of a CO2 laser presenting the flexible delivery system for oral applications.
Source: Dr. Georgios E Romanos.
The optical fiber (Figure 1.10) works by a total internal reflection in which the index of reflection inside the core of the fiber is higher than the index of reflection of the cladding (Ghatak and Thyagarajan 1998). They are currently used in many laser systems, because they are reasonably priced. These light pipes can be quite long, so that the laser unit and the surgical area can be physically separated.
They have a broad clinical application possibility, among others in gastroenterology, vascular surgery, gynecology, and also in dentistry.
A disadvantage of these waveguides is the relative loss of power at high deflection of the fiber and the limitations in focusing. Of particular clinical importance is the intracorporeal application of the fiber, due to the high quartz fiber flexibility. Flexible fibers can nowadays be inserted, by means of endoscopy, in difficult to access areas, and there they can be therapeutically used.
For intracorporeal clinical use an optical fiber is essential. Waveguides are not currently used intracorporeally, as combustion products in the case of not‐with‐window‐closed waveguides can contaminate the inner surfaces. Technological efforts for the optimization of beam control systems are intensively carried out in the various fields of clinical medicine.

Figure 1.10 Flexible optical fibers for medical and dental applications.
Source: Dr. Georgios E Romanos.
1.7 Applicators
Applicators are technical devices of the laser unit, which allow direct transmission of the laser beam in the tissue. They have the shape of a handpiece or a tube (fiber applicator).
1.7.1 Handpieces
Handpieces are primarily used for mirror swivel arms and make possible the transmission and focusing of the beam on a tissue area without contact (noncontact). They can shrink the irradiated area considerably, depending on the manufacturer and by means of special tips made of ceramic or metal (Figures 1.11–1.14). Bent metal spikes can be also used for less accessible regions of the oral cavity. Likewise, there are handpieces with beam deflection.

Figure 1.11 Irradiation of soft tissues in the lamb vestibule using a ceramic tip and CO2 laser (left) and a glass flexible fiber of a diode laser (right). Observe the superficial carbonization of the CO2 laser excision compared to the diode ablation. Different diameter applicators were used (ceramic cylindrical tip for the CO2 and narrow glass fiber for the diode laser).
Source: Dr. Georgios E Romanos.



Figures 1.12–1.14 Special tips for direct connection with the hand piece (left) for Er:YAG laser made by sapphire or glass fiber for Er:YAG or diode lasers (middle) and for Er,Cr:YSGG laser (right).
Source: Dr. Georgios E Romanos.
1.7.2 Fiber Applicators
Fiber applicators are straight or curved tubes that allow contact of the flexible fibers with the tissues. The fiber can be fixed in the applicator by a simple screw. During a contact application, the fiber tip is worn out over time, which reduces the beam profile and decreases the available power density (Figures 1.15 and 1.16). Thus, an optimal ablation is prevented.
A fiber cable consists of the main core (8 μm), the cladding (125 μm) covered by the coating (approx. 250 μm), and the jacket (400 μm), which protects the entire fiber optic.


Figures 1.15 and 1.16 Diode laser irradiation during frenectomy and partial vestibuloplasty using a contact of the fiber tip with the tissues (left). This may allow damage of the tip, decrease of the power density, and, due to overheating, potential scar tissue formation. For this specific indication, initiated tips are strongly recommended (right).
Source: Dr. Georgios E Romanos.

Figure 1.17 Special tools to remove plastic coating around glass fiber without damaging the fiberoptic.
Source: Dr. Georgios E Romanos.
Laser manufacturers have special tools (Figure 1.17) for cutting the glass fibers after removal of the plastic coating (clearing).
With new laser developments, the industry grows, and laser devices become smaller and more powerful every day. An example is the growth of the Er,Cr:YSGG lasers (Biolase Inc.) over the last 20 years demonstrating a significant reduction of the size of the devices.
The spot size is very important since smaller spot sizes are associated with higher fluence. Thermal transfer in small spot sizes can be more effective without damage to the surrounding tissues. In contrast to this, a larger spot size requires higher energy levels or longer irradiation periods, which may have side effects to the surrounding tissues (i.e. carbonization or overheating). In this case, when clinicians try to avoid overheating tissues, the therapeutic energy level cannot be achieved and, therefore, the final outcome is insufficient.
1.8 Laser Types Based on the Active Medium
The currently known laser systems are, according to their active medium, divided into the following types (Table 1.2):
- Gas lasers (He:Ne, CO2, excimer [ArF or KrF] or argon laser)
- Solid state lasers (Nd:YAG, Ho:YAG, Er:YAG, Er,Cr:YSGG, rubin, alexandrite laser)
- Liquid (dye) lasers (containing liquid colorant as the medium, e.g. Rhodamine G6, Coumarin, etc.)
- Semiconductor (diode) lasers, so‐called GaAs, GaAlAs lasers (containing semiconductor as the medium)
- “Free‐electron” lasers (using an electron accelerator, not available for dentistry)
Table 1.2 Laser systems with applications in medicine.
Wavelength (nm) | Laser | Active Medium | Mode | Application |
---|---|---|---|---|
193 | Argon fluoride | ArF | Pulsed | Ophthalmology |
Excimer | ||||
308 | Xenon chloride | XeCl | Pulsed | Vascular surgery |
Excimer | ||||
488 | Argon ion | Ar | CW | Various surgeries |
511 | Copper vapor | Cu ions | Pulsed | Dermatology |
514 | Argon | Ar | CW | Various surgeries |
532 | KTP (frequency | Nd:YAG | Pulsed | Various surgeries |
doubled Nd:YAG) | KTP crystal | |||
627.3 | Gold vapor | Au ions | Pulsed | PDT |
632.8 | Helium‐neon | Neon gas | CW | Biostimulation |
647 | Krypton | Ionized Kr gas | CW | Retinal coagulation |
694.3 | Ruby | Cr3+:Al2O3 | Pulsed | Dermatology |
500–800 | Dye | Dyes | CW/pulsed | PDT, Dermatology |
670–1550 | Diode | Ga‐As | CW/pulsed | various surgeries |
798 | Alexandrite | Crystal | Pulsed | Research |
1064 | Neodymium:YAG | Crystal | Pulsed | Various surgeries |
1070 | Ytterbium:YAG | Crystal | Pulsed | Dermatology |
2010 | Thulium | Tm:YAG crystal | Pulsed | Urology |
2140 | Holmium | ThHoCr:YAG crystal | Pulsed | Cartilage surgery |
2940 | Er:YAG | Er:YAG crystal | Pulsed | Ophthalmology |
10 600 (or 9300) | CO2 | CO2 | CW/pulsed | Various surgeries |
Physically seen, the change of energy levels of electrons in an atom produces the laser radiation. With the gas and solid‐state lasers, the atoms are stimulated by electron collisions. On the contrary, in the excimer, or in the dye lasers, a transition of electrons to molecules takes place.
All lasers used in medicine, including their wavelengths, are in the Table 1.2.
Below are described those types of lasers, which are primarily used in surgical dentistry.
1.8.1 Gas Lasers
Such lasers use gas as the active medium. These lasers are relatively inexpensive and can achieve high power in continuous wave mode. Known systems are the CO2, the argon, the He:Ne, and the excimer laser. In these types of lasers, the active medium is stimulated by an optical pumping mechanism or by electrical discharge. Flowing gas is required, and usually there is no need for gas refill for a long lifetime. Such lasers are the main lasers for general surgery especially the carbon dioxide laser. However, the bulky size of these devices and the fragile construction make these lasers not the first choice of application in private practices.
1.8.1.1 CO2 Laser (10 600 nm)
This type of laser was developed in the US between the early 1960s and the early 1980s. The active medium consists of carbon dioxide (CO2), nitrogen, and helium. The mixing ratio of the laser medium is 4.5% CO2, 13.5% N2, and 82% He and represents a nontoxic gas mixture. Nitrogen molecules are pumped by an external energy source, which by its energy activates the molecules of the active medium (CO2). For this reason, this type of laser is also called a molecular laser.
Depending on the type of discharge, they are currently operated as continuous (CW) or pulsed systems. During the production of the laser light, an excessive overheating of the optical resonator is prevented by the cooling effect of helium. Optical materials for the CO2 laser are, among others used, germanium, zinc selenide, and gallium arsenide. Even the slightest dirt on the lenses can cause destruction.
In terms of CO2 laser construction characteristics, there are different types of CO2 lasers including glass tubes dating back to the 1960s; this technology features a relatively short lifetime and high maintenance costs; it requires up to 20 000 volts and flowing liquid coolant, both of which are expensive in service. Innovative technology with all‐metal tubes was developed in 1990s; it features rugged an all‐metal air‐cooled resonator design, long lifetime (up to 45 000 hours), low cost, low voltage RF transistor‐operated power supplies, and excellent laser pulsing capabilities (Figure 1.18).

Figure 1.18 Innovative all metal‐tube compared to the classic old glass tube of CO2 laser systems
(courtesy: LightScalpel, Inc.).
With a wavelength of 10 600 nm (invisible infrared range), the beam can be well absorbed by the enamel, so that at first it was considered to use this type of laser in the cavity preparation, the conditioning of dental enamel, and treatment of caries (Lobene and Fine 1966; Lobene et al. 1968; Stern et al. 1972). Significant increases in temperature on the tooth surface strongly limited the use of conventional CO2 lasers (CW or pulsed) at the processing of hard tissues (Stewart et al. 1985). On the contrary, Melcer et al. (1984) demonstrated in a clinical trial with 1000 patients positive observations in the removal of caries. In an animal study Melcer et al. (1987) histologically confirmed the formation of secondary dentin and the sterilization of dentin and pulp during the application of CO2 laser.
The absorption of the laser beam increases by water. Since its penetration depth is low (ca. 0.1–0.3 mm) and the surrounding tissue is hardly heated, modern CO2 lasers can be primarily used in the superficial manipulating of soft tissues (Figure 1.19). The coagulating effect on small blood vessels allows a blood‐free and clear surgical field.
1.8.1.2 CO2 Laser (9300 nm)
This is a relatively new development of the CO2 (9300 nm) laser with applications in hard and soft tissues in dentistry. Due to the relatively high absorption by hydroxyapatite, this wavelength can be used for removal of enamel and dentin.
The first laser with FDA clearance for soft and hard tissue applications in dentistry is the SOLEA (Convergent Dental). Compared to the conventional CO2 lasers and the Er:YAG lasers, which vaporize water and enamel, this new laser uses an oxygen‐18 isotope and other modifications to emit 9.3 μm, matching the peak absorption of hydroxyapatite. Therefore, it can be used for removal of decay and also soft tissue excisions with controlled bleeding. Since this wavelength is relatively new in dentistry, more case series and clinical applications are needed to demonstrate the long‐term effects of this wavelength on the tissues.

Figure 1.19 Development of CO2 lasers over time by LightScalpel, Inc. demonstrates the modern and robust design for surgical applications using hollow guide technology. Luxar (MegaPulse, Lightscalpel)
1.8.1.3 Argon‐Laser
The argon laser is an ion laser and is currently not popular in dentistry. Its wavelength is in the visible range of light (488 nm blue or 514.5 nm green light) and its capacity is up to 30 W. Almost all its power is converted into heat, which is why adequate water cooling is necessary.
Initially in the 1960s the argon laser was introduced in gynecology, dermatology, ENT, and ophthalmology. In dentistry, it is useful for caries diagnosis; it reduces the polymerizing time in the therapy with hybrid or micro‐filled composite fillings (Kelsey et al. 1989; Powell et al. 1989; Severin and Maquin 1989; Blankenau et al. 1991a, 1991b; Powell et al. 1995) and can also be used in surgery for the removal of vascular lesions (White et al. 1993) (Figure 1.20).
The high absorption of the argon laser light from hemoglobin, hemosiderin, and melanin allows both intra‐ and extraoral, a complication‐free hemostasis of strongly vascular tissues, and the removal of pigmented lesions (Dixon et al. 1986; Hohenleutner and Landthaler 1990; Kutsch and Blankenau 1995; Poetke et al. 1996). Vessels up to a diameter of 1 mm can be coagulated. The optical penetration depth of the argon laser is limited to about 1 mm. The superficial water cooling allows a doubling of the thermal impact depth by about 2 mm. Thermal damage to the skin can be minimized by the use of saline solution and pressing with a glass spatula (Poetke et al. 1996).

Figure 1.20 Argon laser device (Premier, Irvine, CA).
1.8.1.4 He:Ne Laser
This laser is a neutral atom laser. It contains neon as the active medium and helium as pumping gas at a ratio of 1 : 10. The light is emitted at a wavelength of 633 nm. He:Ne lasers operate in continuous wave mode. The output power is at 0.5 to 50 mW relatively low. Reliability, manageability, and a relatively low price compensate for the low working efficiency of this laser. The He:Ne laser is currently used as a target (the so‐called pilot laser), as well as a laser light pointer in holography. In medicine, it belongs to the group of soft lasers that are used to support wound healing and pain reduction. Further possible applications are found in the counting of cells and measuring of the eye in ophthalmology.
1.8.2 Crystal Lasers
Crystal lasers (usually named as “solid‐state” lasers) are lasers with a crystal as an active medium. Usually, the YAG (yttrium‐aluminum‐garnet) crystal is used in these lasers. Approximately 1% of the yttrium atoms is replaced (“doped”) with neodymium, to have the Nd:YAG (Neodymium: yttrium, aluminum, garnet) laser, which is the most known laser type of this group. The invisible 1064 nm wavelength penetrates deeply into biological tissues, compared to the 532 nm (half of 1064 nm) which penetrates far less and is visible. Such crystals are KTP (potassium triphosphate), producing the frequency‐doubled Nd:YAG (KTP) lasers (green output) with many applications in the treatment of vascularized tissues due to the high absorption by hemoglobin.
Similarly, there are Er:YAG (erbium: yttrium, aluminum, garnet), Er,Cr:YSGG (erbium, chromium:yttrium, scandium, gallium, garnet) and the alexandrite lasers. Less popular crystal dental lasers and some no longer commercially available are the Ho:YAG (holmium: yttrium, aluminum, garnet; 2120 nm), the ruby (694.3 nm), the Nd:CGSGG (neodymium: chromium, gadolinium, scandium, gallium, garnet; 1061 nm) and the Nd:YAP (neodymium: yttrium, aluminum, perovskite; 1340 nm) laser.
These lasers or any glass lasers cannot operate in a CW mode in order to avoid risks of overheating and damage of the laser crystal.
1.8.2.1 Nd:YAG Laser
The Nd:YAG laser was used in dentistry for the first time in 1977 in animal studies in order to test its effect on the pulp (Adrian 1977). Nowadays, it is the most important known solid‐state laser with a wavelength of 1064 nm. In the normal pulse mode, it provides energies up to 50 J, and has as a continuous beam (CW laser) and an output power up to 150 W. It is also used as an industrial laser for material processing (Abdurrochman et al. 2014). However, in dentistry the Nd:YAG laser can be used only in pulsed mode due to the high risk of tissue overheating and deep tissue penetration. The laser beam is absorbed from only a small amount of water and works in contact with the tissue. The heat effect occurs deep in the tissue of the irradiated area and has a strong coagulation effect. This leads to the shrinkage of the tissue, and vessels up to a diameter of 2– 3 mm can be closed. This hemostatic effect of the Nd:YAG laser is used in many ways in clinical surgery. Its biological effects are coagulation, carbonization, and vaporization (Frank 1989).
The application of Nd:YAG laser in medicine was tested by extensive clinical studies and is scientifically validated. One can currently use it in hepatectomy and in the removal of hemorrhoids and highly vascularized tissues, as hemangiomas, without major complications in contact with the tissue used in a fiber optic system (Kiefhaber et al. 1977; Iwasaki et al. 1985; Joffe 1986; Joffe et al. 1986; Poetke et al. 1996).
The first studies in dentistry were carried out by Myers and Myers (1985), and their purpose was the removal of dental caries, concluding that superficial carious enamel lesions can be removed with the Nd:YAG laser. The fine fiber of the Nd:YAG laser system can be used both in the excision of soft tissue, as well as for coagulation (Figures 1.21 and 1.22). In endodontics the positive effect of the laser was shown by means of bacterial reduction in the root canal (Dederich et al. 1984, 1985; Melcer et al. 1987; Hardee et al. 1994; Gutknecht et al. 1996
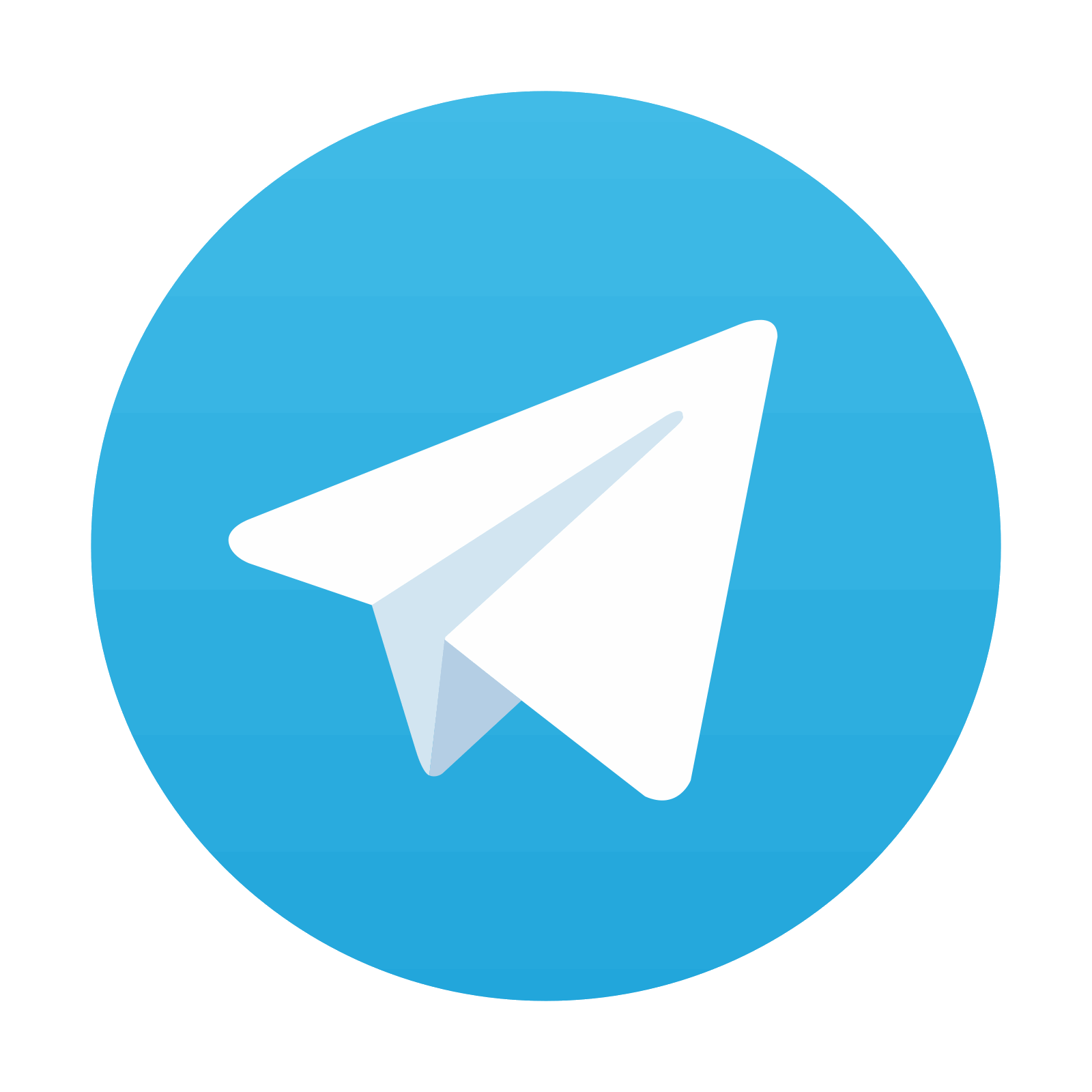
Stay updated, free dental videos. Join our Telegram channel

VIDEdental - Online dental courses
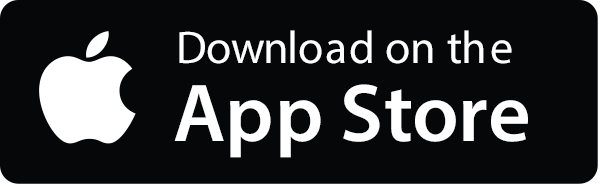
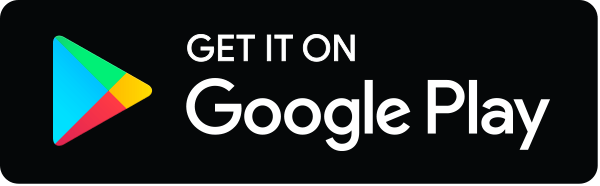