Restorative Materials—Composites and Polymers
The concept of a composite biomaterial, introduced in Chapter 4, can be described as a solid that contains two or more distinct constituent materials or phases when considered at greater than an atomic scale. In these materials, mechanical properties such as elastic modulus are significantly altered in comparison with a homogenous material consisting of either of the phases alone. Enamel, dentin, bone, and reinforced polymers are considered composites, but alloys such as brass are not. The ability to change properties of the macroscale object based on control of the individual constituents is a significant advantage in the use of composite materials.
In dentistry, the term resin composite generally refers to a reinforced polymer system used for restoring hard tissues, for example, enamel and dentin. The proper materials science term is polymer matrix composite or for those composites with filler particles often used as direct-placed restorative composites, particulate-reinforced polymer matrix composite. In this chapter, the term resin composite refers to the reinforced polymer matrix materials used as restorative materials. The class of materials called conventional glass ionomers (GIs) and resin-modified glass ionomers (RMGIs) also are included in the scientific class of composite materials, but because these are water-based materials and have a distinct acid-base setting reaction, they have been traditionally categorized as a class of their own. It is important to remember, however, that most biological materials, including enamel, dentin, bone, connective tissue, muscle, and even cells, are classified as composites within the broad range of biological engineering materials.
Resin composites are used to replace missing tooth structure and modify tooth color and contour, thus enhancing esthetics. A number of commercial resin composites are available for various applications. Traditionally some have been optimized for esthetics and others were designed for higher stress bearing areas. More recently nanocomposites have become available, which are optimized for both excellent esthetics and high mechanical properties for stress bearing areas. Glass ionomers and resin-modified glass ionomers are used selectively as filling materials, usually for small lesions, especially where one or more margins are in dentin and in areas of caries activity.
Resin-based composites were first developed in the early 1960s and provided materials with higher mechanical properties than acrylics and silicates, lower thermal coefficient of expansion, lower dimensional change on setting, and higher resistance to wear, thereby improving clinical performance. Early composites were chemically activated; the next generation were photo-activated composites initiated with ultraviolet (UV) wavelengths. These were later replaced by composites activated in the visible wavelengths. Continued improvements in composite technology have resulted in the modern materials with excellent durability, wear-resistance, and esthetics that mimic the natural teeth. In particular, the incorporation of nanotechnology in controlling the filler architecture has made dramatic improvements in these materials. Moreover, the development of bonding agents for bonding composites to tooth structure (see Chapter 13) has also improved the longevity and performance of composite restorations. A classification of preparation type and recommended composite category is listed in Table 9-1. Characteristics of these composite categories are summarized in Table 9-2.
TABLE 9.1
Types of Restorations and Recommended Resin Composites
Type of Restoration | Recommended Resin Composite |
Class 1 | Multipurpose, nanocomposite, packable microfilled (posterior),∗ compomer (posterior)∗ |
Class 2 | Multipurpose, nanocomposite, packable, laboratory, microfilled (posterior),∗ compomer (posterior)∗ |
Class 3 | Multipurpose, nanocomposite, microfilled, compomer |
Class 4 | Multipurpose, nanocomposite |
Class 5 | Multipurpose, nanocomposite, microfilled, compomer |
Class 6 (MOD) | Packable, nanocomposite |
Cervical lesions | Flowable, compomer |
Pediatric restorations | Flowable, compomer |
3-unit bridge or crown | Laboratory (with fiber reinforcement) |
Alloy substructure | Laboratory (bonded) |
Core build-up | Core |
Temporary restoration | Provisional |
High caries-risk patients | Glass ionomers, hybrid ionomers (see Chapter 8) |
∗Special microfilled composites and compomers are available for posterior use.
TABLE 9.2
Characteristics of Various Types of Resin Composites
< ?comst?>
Type of Composite | Size of Filler Particles (µm) | Volume of Inorganic Filler (1%) | Handling Characteristics and Properties | |
Advantages | Disadvantages | |||
Multipurpose | 0.04, 0.2-3.0 | 60-70 | High strength, high modulus | |
Nanocomposite | 0.002-0.075 | 78.5 | High polish, high strength, high modulus | |
Microfilled | 0.04 | 32-50 | Best polish, best esthetics | Higher shrinkage |
Packable | 0.04, 0.2-20 | 59-80 | Packable, less shrinkage, lower wear | |
Flowable | 0.04, 0.2-3.0 | 42-62 | Syringeable, lower modulus | Higher wear |
Laboratory | 0.04, 0.2-3.0 | 60-70 | Best anatomy and contacts, lower wear | Lab cost, special equipment, requires resin cement |
< ?comen?>< ?comst1?>
< ?comst1?>
< ?comen1?>
Glass ionomers (GIs) were also developed in the 1960s and are based on an acid-base cement-forming reaction between fluoroaluminosilicate (FAS) glass powder, similar to those used in silicate cements, and aqueous solution of polycarboxylic acids. These were less prone to dissolution than silicates, but the early materials suffered from difficulty of manipulation, technique sensitivity, and poor esthetics. Advances in these materials have continued and the modern materials have improved properties. Resin-modified glass ionomers (RMGIs) were invented in the late 1980s to preserve the advantages of fluoride release and clinical adhesion of the conventional GIs yet provide the ease of light-curing and good esthetics of resin-based materials. The use of nanotechnology in resin-modified glass ionomer has resulted in enhanced esthetics of these materials.
Multipurpose Composites
Composition
Overview and Classification
A resin composite is composed of four major components: organic polymer matrix, inorganic filler particles, coupling agent, and the initiator-accelerator system. The organic polymer matrix in most commercial composites today is a cross-linked matrix of dimethacrylate monomers. The most common monomers are aromatic dimethacrylates. The double bonds at each end of these molecules undergo addition polymerization by free-radical initiation. Although these monomers can provide an optimum of optical, mechanical, and clinical properties, they are rather viscous and have to be blended with low-molecular-weight diluents monomers so that a clinically workable consistency may be obtained upon incorporation of the fillers. More recently low-shrink composites have been introduced that contain, for example, monomers with epoxy (also known as oxirane) functional groups at the ends. The polymerization of these monomers is initiated by cations. Other commercial resin composites utilize various monomers and filler technology to reduce polymerization shrinkage or stress.
The dispersed inorganic filler particles may consist of one or more inorganic materials such as finely ground quartz or glass, sol-gel derived ceramics, microfine silica, or more recently nanoparticles. Two-dimensional diagrams of fine and microfine particles surrounded by polymer matrix are shown in Figure 9-1.
The coupling agent, an organosilane (often referred to as silane), is applied to the inorganic particles by the manufacturer to surface-treat the fillers before being mixed with the unreacted monomer mixture. Silanes are called coupling agents, because they form a bond between the inorganic and organic phases of the composite. One end of the molecule contains functional groups (such as methoxy), which hydrolyze and react with the inorganic filler and the other end has a methacrylate double bond that copolymerizes with the monomers.
The role of the initiator-accelerator system is to polymerize and cross-link the system into a hardened mass. The polymerization reaction can be triggered by light-activation, self-curing (chemical activation), and dual curing (chemical and light-curing).
Resin Matrix
Methacrylate Monomers
The vast majority of monomers used for the resin matrix are dimethacrylate compounds. Two monomers that have been commonly used are 2,2-bis[4(2-hydroxy-3-methacryloxy-propyloxy)-phenyl] propane (Bis-GMA) and urethane dimethacrylate (UDMA). Both contain reactive carbon double bonds at each end that can undergo addition polymerization initiated by free-radical initiators. The use of aromatic groups affords a good match of refractive index with the radiopaque glasses and thus provides better overall optical properties of the composites. A few products use both Bis-GMA and UDMA monomers.
The viscosity of the monomers, especially Bis-GMA, is rather high and diluents must be added, so a clinical consistency can be reached when the resin mixture is compounded with the filler. Low-molecular-weight compounds with difunctional carbon double bonds, for example, triethylene glycol dimethacrylate (TEGDMA), or Bis-EMA6 shown below, are added by the manufacturer to reduce and control the viscosity of the compounded composite.
Low-Shrink Methacrylate Monomers
A variety of other methacrylate monomers have been used in the newer commercial products introduced since 2008 for controlling the volumetric
shrinkage and polymerization stress of composites. The general approach relies on increasing the distance between the methacrylate groups resulting in lower cross-link density or increasing the stiffness of the monomers. Some examples include the use of dimer acids, incorporation of cycloaliphatic units, and photocleavable units to relieve stress after polymerization.
The bonding agents widely used with these composites are also prepared from similar organic monomers so that they are compatible with the composites.
Low-Shrink Silorane Monomer
A new monomer system called silorane has been developed to reduce shrinkage and internal stress build-up resulting from polymerization. The name silorane was coined from its chemical building blocks siloxane and oxirane (also known as epoxy). The siloxane functionality provides hydrophobicity to the composite. The oxirane functionalities undergo ring-opening cross-linking via cationic polymerization. Special initiator systems are required for the polymerization of the siloranes (described in the intiator section). Care has to be taken in choosing the filler system. If the filler surface has any residual basicity (as with some glasses and sol-gel derived systems), the composite may become unstable. Furthermore, a specific adhesive system has to be used for bonding these materials during clinical placement.
Fillers and Classification of Composites
Fillers make up a major portion by volume or weight of the composite. The function of the filler is to reinforce the resin matrix, provide the appropriate degree of translucency, and control the volume shrinkage of the composite during polymerization. Fillers have been traditionally obtained by grinding minerals such as quartz, glasses, or sol-gel derived ceramics. Most glasses contain heavy-metal oxides such as barium or zinc so that they provide radiopacity for visualization when exposed to x-rays. It is advantageous to have a distribution of filler diameters so that smaller particles fit into the spaces between larger particles and provide more efficient packing. Recently, nanofillers have been introduced into composites.
These are described in the section on Nanofillers and Nanocomposites.
A helpful method of classifying dental composites is by the particle size, shape, and the particle-size distribution of the filler. This classification is presented below.
Macrofills
The early composites were macrofills. These composites contained large spherical or irregular shaped particles of average filler diameter of 20 to 30 μm. The resultant composites were rather opaque and had low resistance to wear.
Hybrid and Microhybrid Composites
The hybrid composites are two types of fillers that are blended together: (1) fine particles of average particle size 2 to 4 μm and (2) 5% to 15% of microfine particles, usually silica, of particle size 0.04 to 0.2 μm. In microhybrid composites, the fine particles of a lower average particle size (0.04 to 1 μm) are blended with microfine silica. The fine particles may be obtained by grinding glass (e.g., borosilicate glass, lithium or barium aluminum silicate glass, strontium or zinc glass), quartz, or ceramic materials and have irregular shapes. The distribution of filler particles provides efficient packing so that high filler loading is possible while maintaining good handling of the composite for clinical placement. Microhybrid composites may contain 60% to 70% filler by volume, which, depending on the density of the filler, translates into 77% to 84% by weight in the composite. Most manufacturers report filler concentration in weight percent (wt%). A micrograph of a typical, fine glass filler is shown in Figure 9-2, A. Hybrids and microhybrids have good clinical wear resistance and mechanical properties and are suitable for stress-bearing applications. However, they lose their surface polish with time and become rough and dull.
Nanocomposites
Recently the incorporation of nanotechnology into designing and manufacturing of composites has greatly improved their properties. Nanocomposites describe this class of composites. The nanofiller technology is described in the next section.
Nanofillers and Nanocomposites
The latest advancement in composite technology has been the use of nanotechnology in development of fillers. Nanotechnology is the production of functional materials and structures in the range of 1 to 100 nanometers (nm)—the nanoscale—by various physical and chemical methods. Nanotechnology requires devices and systems to create structures that have novel properties and functions because of their small sizes. Thus it implies the ability to control and manipulate structures at the atomic and/or molecular scale. Although true nanocomposites should have all filler particles in the nanometer size, the term nanotechnology has some hype associated with it, and it is sometimes misused in describing a material. To date oxide nanoparticles have been the most prevalent types of nanomaterials used in dental composites. At present there are two distinct types of dental composites available that contain nanoparticles:
< ?mpslid E67?>< ?mpslid S68?>
< ?mpslid E68?>
Nanofill Composites
All filler particles of true nanofilled composites are in the nanometer range. There are several purposes for incorporating nanofillers in dental composites. First, the size of nanomeric particles is below that of visible light (400-800 nm), which provides the opportunity of creating highly translucent materials. In addition the surface area to volume ratio of the nanoparticles is quite large. The sizes of the smallest nanoparticles approach those of polymer molecules so they can form a molecular scale interaction with the host resin matrix.
Two types of nanoparticles have been synthesized and used for preparing this class of composite. The first type consists of nanomeric particles that are essentially monodisperse nonaggregates and nonagglomerated particles of silica or zirconia. The surface of the nanoparticles are treated with silane coupling agents that allow them to be bonded to the resin matrix when the composite is cured after placement. Nanomers are synthesized from sols, creating particles of the same size. Because of this, if nanomeric particles alone are used to make highly filled composites, the rheological properties are rather poor. To overcome this disadvantage, one manufacturer has designed a second type of nanofiller, which is called nanocluster. The nanoclusters are made by lightly sintering nanomeric oxides to form clusters of a controlled particle size distribution. Nanoclusters have been synthesized from silica sols alone as well as from mixed oxides of silica and zirconia. The primary particle size of the nanomers used to prepare the clusters range from 5 to 75 nm.
It is important to remember that in a nanocluster, the nanoparticles still maintain their individual form, much as in a cluster of grapes. The clusters can be made to have a wide size distribution ranging from 100 nm to submicron level and have an average size of 0.6 micrometers. Figure 9-3, A, shows a scanning electron microscopy (SEM) image of a nanocluster of silica in a commercial composite after the resin matrix was removed by washing with acetone. In this material the surface of the nanoclusters are treated with a silane coupling agent to provide compatibility and chemical bonding with the resin system. Figure 9-3, B, shows the micrograph image of a nanocluster comprised of silica and zirconia. The differences in particle architecture of nanomers, nanoclusters, and conventional microhybrid fillers are readily apparent in transmission electron micrographs (TEMs) of composites prepared from these fillers. Figure 9-4, A, shows the TEM of a nanocomposite filled with 75-nm diameter nanoparticles only; Figure 9-4, B, is that of a nanocomposite filled with a mixture of nanoclusters alone, and Figure 9-4, C, is of a conventional microhybrid composite. To date there is only one true nanofilled dental composite available. In this manufactured composite, a combination of nanomeric particles and nanoclusters are used in optimum combinations. Figure 9-5, A, shows a schematic diagram of this nanocomposite containing a blend of nanocluster and nanomeric fillers and Figure 9-5, B, shows a TEM of the nanocomposite showing the presence of the two types of nanofillers.
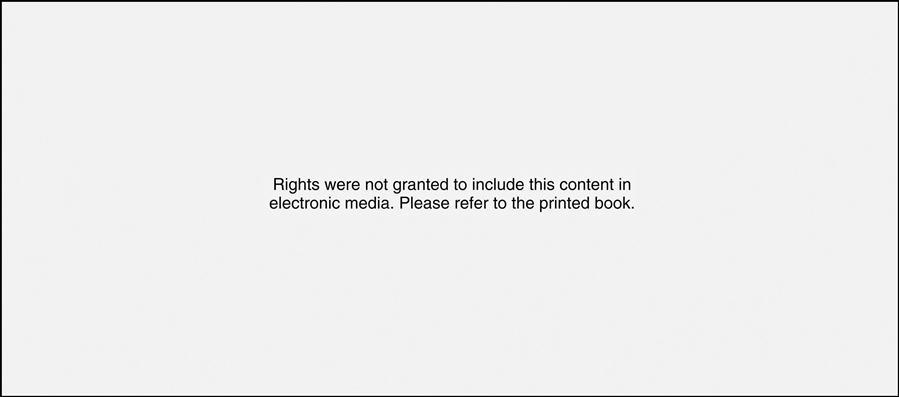
Rights were not granted to include this figure in electronic media. Please refer to the printed book. (A, B, and C from Mitra SB, Wu D, Holmes BN: J. Am. Dent. Assoc. 134, 1382, 2003.)
The uniqueness of the nanofilled composite is that it has the mechanical strength of a microhybrid but at the same time retains smoothness during service like a microfill. The initial gloss of many restoratives is quite good, but in hybrid composites (microhybrids, nanohybrids) plucking of the larger fillers causes loss of gloss. In contrast, in the nanofilled composite, the nanoclusters shear at a rate similar to the surrounding matrix during abrasion. This allows the restoration to maintain a smoother surface for long-term polish retention. Optical analysis of the polish retention can be done using atomic force microscopy after extended toothbrush abrasion.
Nanofillers also offer advantages in optical properties. In general, it is desirable to provide low visual opacity in unpigmented dental composites. This allows for the creation of a wide range of shades and opacities so the clinician can design a highly esthetic restoration. In hybrid types of composites the filler particles are 0.4-3.0 μm in size. When particles and resin are mismatched in the refractive index, which measures the ability of the material to transmit light, the particles will scatter light and produce opaque materials. Nanomeric fillers particles are far smaller than the wavelength of light, making them unmeasurable by refractive index. When light enters, long wavelength light passes directly through and materials show high translucency. As shown by Figure 9-6, the discs made with hybrid and microfill fillers are rather opaque. The nanofill composite sample made predominantly with the nanomeric filler is quite clear; the background can be easily seen through the composite. In addition, when placed on a black background, the nanomeric and nanocluster particles preferentially scatter blue light, giving the composite an opalescent effect. This gives a more life-like appearance that matches natural enamel, which also exhibits the same effect.
The ability to create a nanocomposite with very low opacity provides the ability to formulate a vast range of shade and opacity options from the very translucent shades needed for the incisal edge and for the final layer in multilayered restorations to the more opaque shades desired in the enamel, body, and dentin shades. This allows the clinician the flexibility of choosing a single shade or a multishade layering technique depending on the esthetic needs. The wear resistance of this material after 3 and 5 years of clinical use was found to be similar to human enamel.
Nanohybrid Composite
Several manufacturers have placed nano-sized particles in their microhybrids. These composites have been described as nanohybrids. Because the smoothness and wear of any composite is often determined by the size of its largest filler particles as with microhybrids, the surface of nanohybrids becomes gradually dull after a few years of clinical service.
Interfacial Phase and Coupling Agents
For a composite to have successful clinical performance, a good bond must form between the inorganic filler particles and the organic resin matrix during setting. This is achieved through the use of compounds called coupling agents, the most common of which are organic silicon compounds called silane coupling agents. The surface of the filler is treated with a coupling agent during the manufacture of the composite. A typical silane coupling agent is 3-methacryloxypropyltrimethoxysilane (MPTS), the chemical structure of which is shown below.
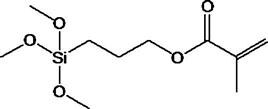
In the low-shrink silorane composite, an epoxy functionalized coupling agent, 3-glycidoxypropyltrimethoxysliane, is used to bond the filler to the oxirane matrix.
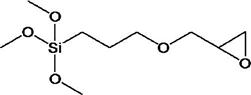
During the filler treatment process, the methoxy groups hydrolyze to generate hydroxyl groups through an acid or base catalyzed reaction. These hydroxyl groups then undergo condensation with the hydroxyl groups on the surface of the filler and become attached by covalent bonds (see the following schematic sketch). Condensation is also possible with the adjacent −OH groups of the hydrolyzed silanes or with water absorbed on the surface of the filler. This results in the formation of a very thin mono- or multilayer polymeric film on the surface of the filler with unreacted double bonds. During the curing of the composite, the double bonds of the methacryloxy groups of the treated surface co-reacts with the monomer resins. The coupling agent plays a critical role in the composite. Its functions are summarized below:
• It forms an interfacial bridge that strongly binds the filler to the resin matrix.
< ?mpslid E69?>< ?mpslid S70?>
< ?mpslid E70?>< ?mpslid S71?>
< ?mpslid E71?>< ?mpslid S72?>
• It provides a hydrophobic environment that minimizes water absorption of the composite.
< ?mpslid E72?>
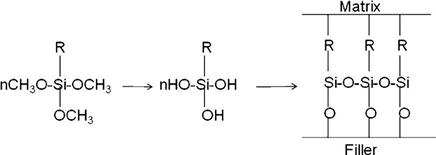
Initiators and Accelerators
The curing of composites is triggered by light or a chemical reaction, with the former being more common. Light activation is accomplished with blue light at a peak wavelength of about 465 nm, which is absorbed usually by a photo-sensitizer, such as camphorquinone, added to the monomer mixture during the manufacturing process in amounts varying from 0.1% to 1.0%.
In methacrylate composites, free radicals are generated upon activation. The reaction is accelerated by the presence of an organic amine. Various amines have been used, both aromatic and aliphatic. Examples of two such amines are shown below. The amine and the camphorquinone are stable in the presence of the oligomer at room temperature, as long as the composite is not exposed to light. Although camphorquinone is the most common photo-sensitizer, others are sometimes used to accommodate special esthetic considerations. Camphorquinone adds a slight yellow tint to the uncured composite paste. Although the color bleaches during cure, sometimes clinicians find shade matching difficult with the color shift.
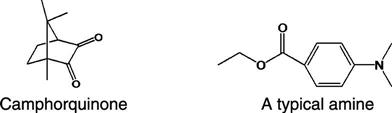
Chemical activation is accomplished at room temperature by an organic amine (catalyst paste) reacting with an organic peroxide (universal paste) to produce free radicals, which in turn attack the carbon double bonds, causing polymerization. Once the two pastes are mixed, the polymerization reaction proceeds rapidly.
Some composites, such as core and provisional products, are dual cured. These formulations contain initiators and accelerators that allow light activation followed by self-curing or self-curing alone.
In the silorane composite, the initiator system generates cations when irradiated with light. One of the components is the camphorquinone photo-sensitizer enabling the composite to be cured by a dental curing unit. Other components of the initiation system are iodonium salts and electron donors, which generate the reactive cationic species that start the ring opening polymerization process.
Pigments and Other Components
Inorganic oxides are usually added in small amounts to provide shades that match the majority of tooth shades. The most common pigments are oxides of iron. Numerous shades are supplied, ranging from very light shades to yellow to gray. Various color scales are used to characterize the shades of the composites. A UV absorber may be added to minimize color changes caused by oxidation. Darker and more opaque shades of composites cannot be cured to the same depth as the lighter translucent shades.
Fluorescent agents are sometimes added to enhance the optical vitality of the composite and mimic the appearance of natural teeth. These are dyes or pigments that absorb light in the ultraviolet and violet region (usually 340-370 nm) of the electromagnetic spectrum, and re-emit light in the blue region (typically 420-470 nm). These additives are often used to enhance the appearance of color causing a perceived “whitening” effect, making materials look less yellow by increasing the overall amount of blue light reflected.
Polymerization Reactions
Polymerization of Methacrylate Composites
Methacrylate composites form the workhorse of direct restorative materials. The polymer network of these composites are formed by a process called free-radical addition polymerization of the corresponding methacrylate monomers. The polymerization reaction takes place in three stages: initiation, propagation, and termination and is shown in the following scheme.

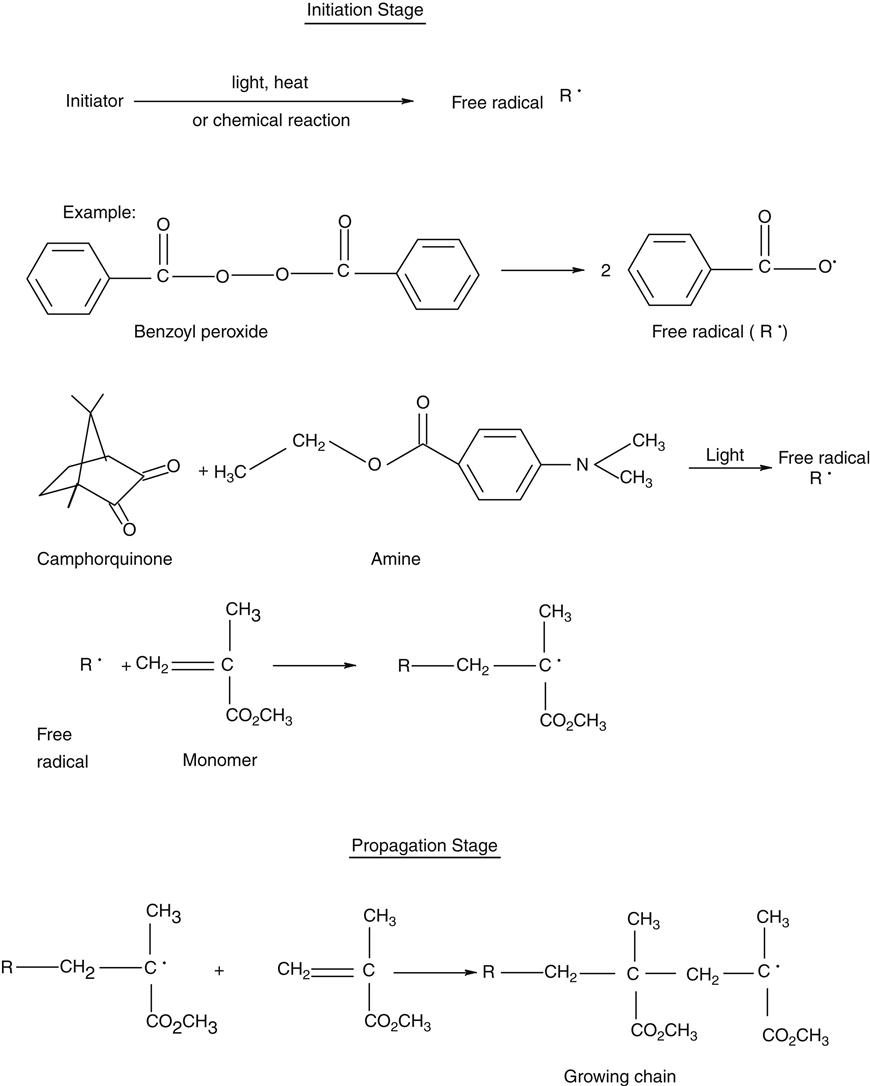
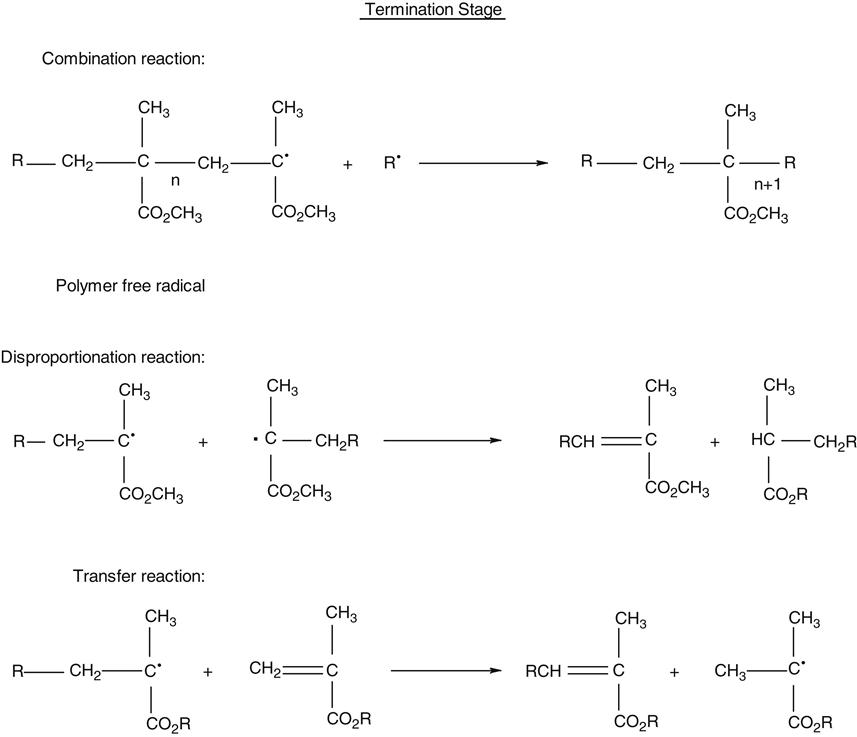
The polymerization reaction of self-cured composites is chemically initiated at room temperature with a peroxide initiator and an amine accelerator. Polymerization of light-cured composites is triggered by visible blue light. The photo-initiators used are described in the section on Initiators and Accelerators. Dual-cured products use a combination of chemical and light activation to carry out the polymerization reaction. At this stage, an active free radical species, designated as R• in the foregoing scheme, is first formed as the initiating species. This free radical adds to a monomer species generating an active center monomer radical.
The initiation stage is followed by the propagation stage during which rapid addition of other monomers molecules to the active center occurs to provide the growing polymer chain. The propagation reaction continues to build molecular weight and cross-link density until the growing free radical is terminated. The termination stage may take place in several ways as indicated, where n represents the number of mer units.
The polymerized resin is highly cross-linked because of the presence of difunctional carbon double bonds. The degree of polymerization varies, depending on whether it is in the bulk or in the air-inhibited layer of the restoration. Polymerization of light-cured composites varies by the distance of the light from the restoration and the duration of light exposure. The percentage of double bonds that react may vary from 35% to 80%. The degree of polymerization is higher for laboratory composites that are post-cured at elevated temperatures and light intensities.
During polymerization, molecules have to approach their “neighbors” to form chemical bonds with them. Reduction of volume, or shrinkage, is generally observed during polymerization because two factors are reduced: the Van der Waals volume and the free volume. The Van der Waals volume is the volume of molecule itself derived from the atoms and bond lengths. Reduction in the Van der Waals volume takes place during polymerization because of a change in the bond lengths (conversion of double bonds to single bonds). The free volume of a molecular species, whether a monomer or a polymer, is the volume occupied by it due to its random rotational and thermal movement. When monomers are converted to polymers, reduction of the free volume occurs because the rotation of the polymer chain is more restricted than in unpolymerized monomer molecules. A schematic illustration of the polymerization of methacrylate resin resulting in shrinkage is shown in Figure 9-7.
Manufacturers have taken several steps to minimize the polymerization contraction in methacrylate composites by one or more of the following methods:
• Filling the monomer resins with prepolymerized resins
< ?mpslid E73?>< ?mpslid S74?>
• Maximizing the amount of inorganic filler
< ?mpslid E74?>< ?mpslid S75?>
• Using high molecular mass methacrylate monomers
< ?mpslid E75?>
In addition, incremental placement of methacrylate composites in the tooth cavity, necessitated by their limited depth of cure, controls shrinkage stress so that clinical success of the modern-day methacrylate composite is quite excellent.
Polymerization of Silorane Composites
Polymerization of the silorane molecule takes place through a cationic initiation process during which the oxirane segments undergo ring opening to form covalent single bonds with their neighbors. The chemistry of the polymerization is shown below:
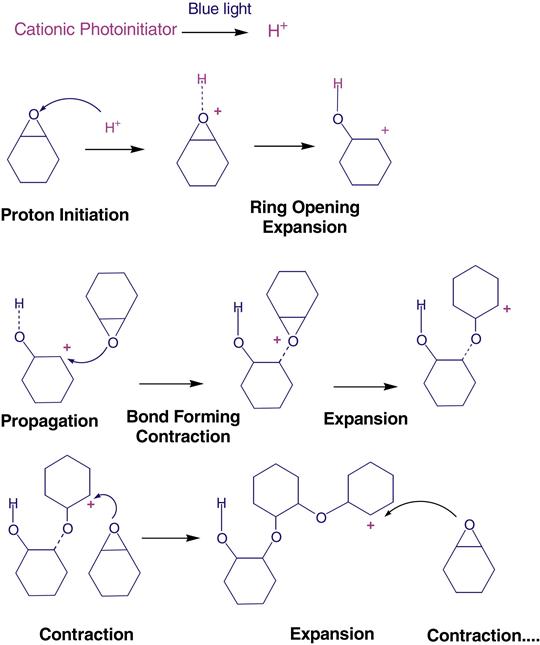
The ring-opening chemistry of the siloranes starts with the cleavage and opening of the oxirane ring. This process gains space and counteracts some of the reduction in volume when chemical bonds are established to form the polymer. A schematic diagram to depict the polymerization is shown in Figure 9-8.
The silorane composites generate lower volume shrinkage and stress upon polymerization. It is still important to place these composites in increments because of limited depth of cure. In addition, special adhesives are needed.
Packaging of Composites
Composites as supplied from the manufacturer are in their pre-cured state and hence have to be packaged with adequate protection against inadvertent setting. The primary package is made from a plastic material that allows the diffusion of oxygen (which works as an inhibitor for methacrylate polymerization) and also prevents moisture absorption in humid environments.
Light-Cured Composites
Because the setting of light-cured composite is triggered by visible light, these materials have to be protected from premature curing when supplied from the manufacturer and stored in the dental office. Hence they are packaged in opaque, most often black, plastic syringes or unit-dose capsules, sometimes referred to as compules. In the latter case, a delivery gun for direct intra-oral placement is provided. An advantage of the capsule delivery is the lower risk of cross-infection. Composite packaging and delivery systems are shown in Figure 9-9. The composites are supplied in a variety of shades and opacities. Some manufacturers color code their capsule caps or syringe plungers for ease of identification of the shades and/or opacities.
Self-Cured and Dual-Cured Composites
Self-cured and dual-cured materials are supplied in two syringes. Two pastes are mixed to initiate the chemical cure. An example of a two-paste core build-up composite is shown in Figure 9-10. It is advisable to store these materials in a cool temperature to prolong their shelf-life.
Properties of Composites
Overview
In order to have good clinical service life, composites have to meet certain performance criteria. Important physical, mechanical, and clinical properties are described in this section. Selected properties of various types of composites are listed in Table 9-3. Values of properties for polymer-based filling and restorative materials based on ISO 4049 (ANSI/ADA No. 27) are summarized in Table 9-4.
TABLE 9-3
< ?comst?>
Property | Nano-composite∗ | Multipurpose Composite | Microfill Composite | Packable Composite | Flowable Composite | Laboratory Composite | Core Composite | Conventional Glass Ionomer | Resin-modified Glass Ionomer |
Flexural strength (MPa) | 180 | 80-160 | 60-120 | 85-110 | 70-120 | 90-150† | ― | 7–15 | 50-60 |
Flexural modulus (GPa) | ― | 8.8-13 | 4.0-6.9 | 9.0-12 | 2.6-5.6 | 4.7-15† | ― | ― | ― |
Flexural fatigue limit (MPa) | ― | 60-110 | ― | ― | ― | ― | ― | ― | ― |
Compressive strength (MPa) | 460 | 240-290 | 240-300 | 220-300 | 210-300 | 210-280 | 210-250 | 10–15 | 200-250 |
Compressive modulus (GPa) | ― | 5.5-8.3 | 2.6-4.8 | 5.8-9.0 | 2.6-5.9 | ― | 7.5-22 | 7.2-10.3 | 3.2-6.9 |
Diametral tensile strength (MPa) | 81 | 30-55 | 25-40 | ― | 33-48 | ― | 40-50 | 7–15 | 30–40 |
Linear polymerization shrinkage (%) | ― | 0.7-1.4 | 2–3 | 0.6-0.9 | ― | ― | ― | ― | ― |
Color stability, accelerated aging–450 kJ/m2(ΔE∗)‡ | ― | 1.5 | ― | ― | 15 | 1.1-2.3 | ― | ― | ― |
Color stability, stained by juice/tea (ΔE∗)‡ | ― | 4.3 | ― | ― | ― | 1.7-3.9 | ― | ― | ― |
< ?comen?>< ?comst1?>
< ?comst1?>
< ?comen1?>
< ?comst1?>< ?comen1?>∗< ?comst1?>< ?comen1?>Mitra SB, Wu D, Holmes BN: J Am Dent Assoc 134:1382, 2003.
< ?comst1?>< ?comen1?>†< ?comst1?>< ?comen1?>Without fiber reinforcement.
< ?comst1?>< ?comen1?>‡< ?comst1?>< ?comen1?>ΔE <3.3 is considered not clinically perceptible.
TABLE 9.4
Requirements for Polymer-Based Filling and Restorative Materials Based on ISO 4049
< ?comst?>
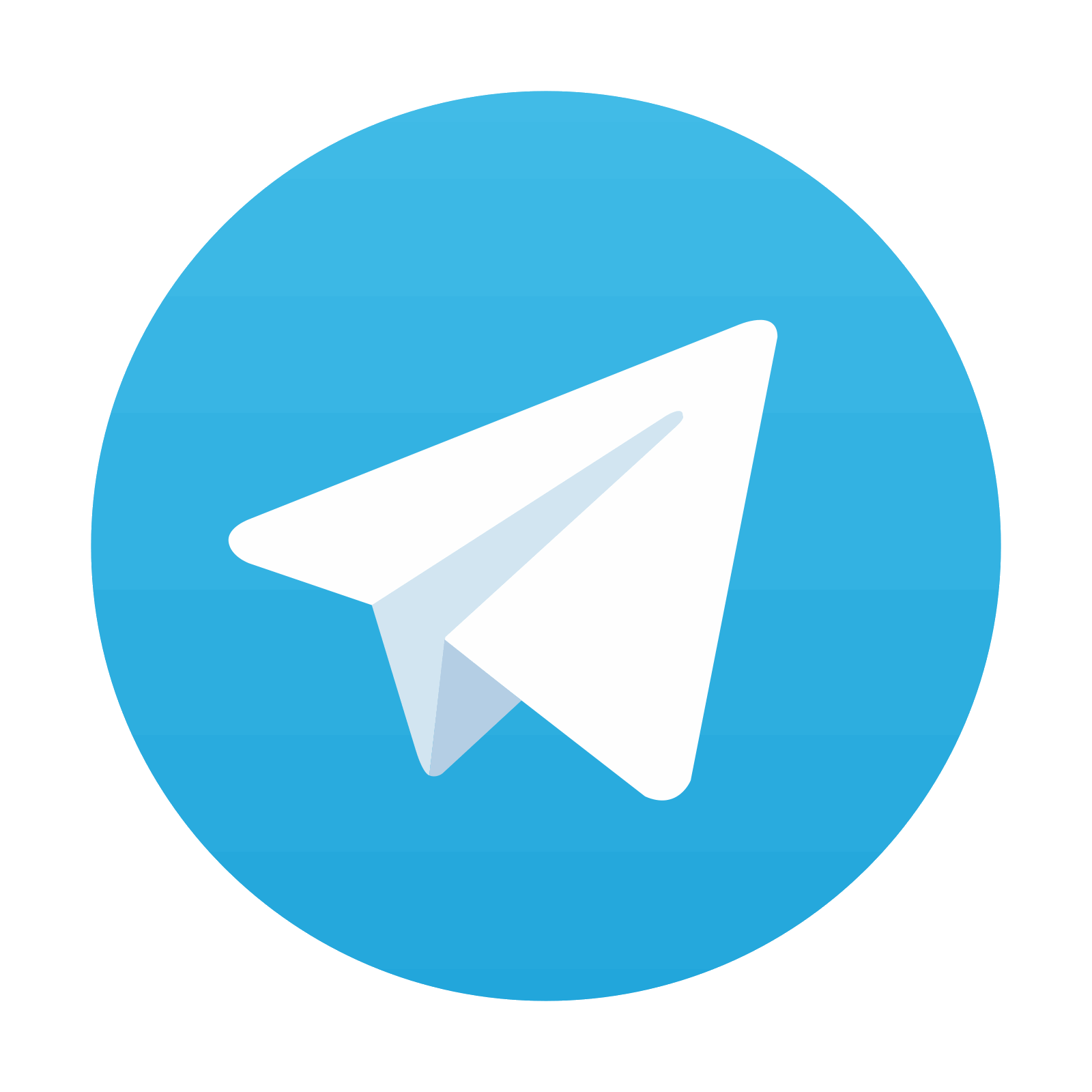
Stay updated, free dental videos. Join our Telegram channel

VIDEdental - Online dental courses
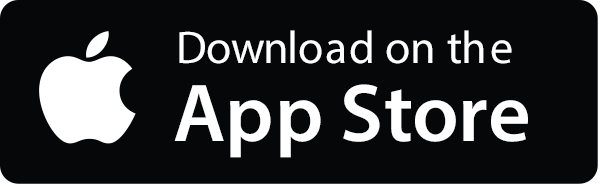
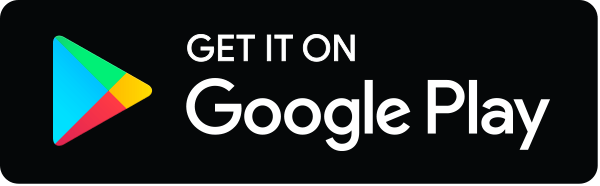