Dental and Orofacial Implants
The practice of restorative dentistry seeks to replace the form and function of missing tooth structure. It was therefore expected that dentistry would follow orthopedic medicine in the use of implants to anchor prosthetic devices and as expected, that has happened. In 2010, the global implant market was $3.2 billion. Sales in 2015 is expected to reach nearly $4.2 billion at a compounded annual growth rate of 6%. In 2010, Europe exhibited a 42% market share. By 2015, the Asia-Pacific region will have the highest annual growth rate.
Dental implants are fixtures that serve as replacements for the root of a missing natural tooth. Implants may be placed in the mandible or maxilla. When properly designed and placed, dental implants bond with bone over time and serve as an anchor for dental prostheses. Dental implants are used to replace a single missing tooth or many teeth, or to support a complete removable denture.
Worldwide, modern single-tooth implants have a success rate of nearly 95% survival at 15 years. Implants are permanent devices, surgically anchored in the oral cavity, that often provide significant advantages over other fixed or removable prosthodontic options. Implants are often more conservative than traditional fixed partial dentures because they conserve tooth structure by eliminating the need for reduction of adjacent abutment teeth and they support the maintenance of healthy bone in the region.
Classification
Historically, dental implants have been classified according to their design. This design was in turn based on the way in which they are surgically implanted. The three types of implants commonly used for the past 40 years are the subperiosteal implant, the transosteal implant, and the endosseous implant (Table 15-1).
TABLE 15.1
Implant Design Classification Scheme
< ?comst?>
Implant Design | Contact with Bone | Composition | Location Used |
Subperiosteal | Directly on bone surface under the gingival tissues; no bone penetration | Co-Cr-Mo (Vitallium) | Maxilla and mandible |
Transosteal | Completely through the bone, penetrating the cortical wall twice | Titanium or Ti-alloy | Mandible only |
Endosteal | Within the bone, penetrating the cortical wall once | Titanium or Ti-alloy | Maxilla and mandible |
< ?comen?>< ?comst1?>
< ?comst1?>
< ?comen1?>
Endosseous Implant
Endosseous implants are by far the most common type of implant placed today. Implants are placed directly into the mandible or maxilla (Figure 15-1). A pilot hole is drilled into the alveolar or basal bone beneath (in cases in which the alveolar bone has been partially or completely resorbed), and the implant body is inserted into this site. The top of the implant is positioned so that it either protrudes slightly through the cortical plate or is flush with the surface of the bone. Typically a superstructure containing a prosthetic tooth or teeth connects to the implant body through an abutment that is screwed into the body directly through the mucosa.
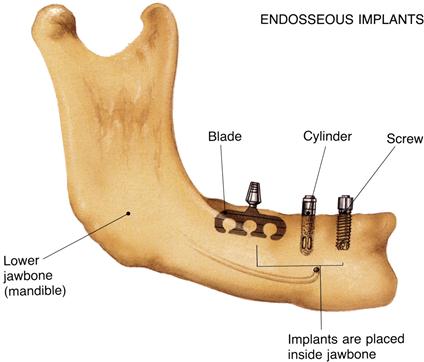
Shown here are three different endosseous implant designs. Notice that all of the designs are implanted directly within the bone. Although the blade design has fallen out of use, the cylinder and screw-shaped versions continue to be the most widely placed implant designs in use today.
Osseointegration and Biointegration
A major issue for implant design is the development of materials that are physically and biologically compatible with alveolar bone. Ideally, bone should integrate with the material, substance, or device and remodel the bone structure around it, rather than responding to the material as a foreign substance by encapsulating it with fibrous tissue. Under optimum circumstances, bone differentiation occurs directly adjacent to the material (osseointegration). Ideally, this osseointegration provides a stable bone-implant connection that can support a dental prosthesis and transfer applied loads without concentrating stresses at the interface between bone and the implant.
Osseointegration is now formally defined as the close approximation of bone to an implant material (Figure 15-2). To achieve osseointegration, the bone must be viable, the space between the bone and implant must be less than 10 nm and contain no fibrous tissue, and the bone-implant interface must be able to survive loading by a dental prosthesis. In current practice, osseointegration is an absolute requirement for the successful implant-supported dental prosthesis. To achieve osseointegration between an implant and bone, a number of factors must be correct. The bone must be prepared in a way that does not cause necrosis or inflammation. The implant must be allowed to heal for a time without a load. Finally, the proper material must be implanted, because not all materials will promote osseointegration.
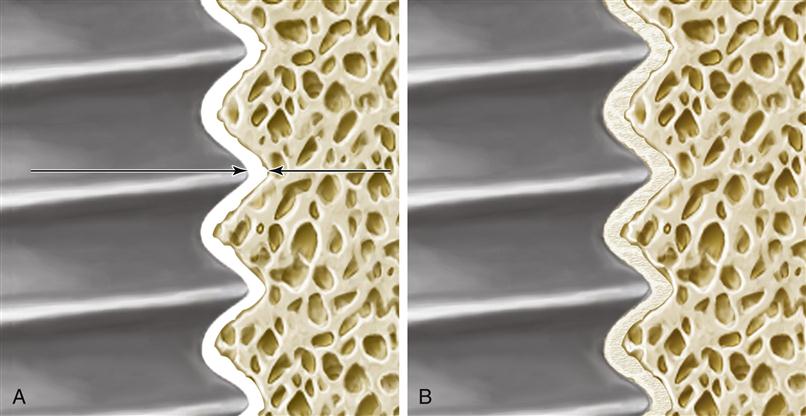
A, In osseointegration, the implant material (left) and the bone (right) closely approximate one another. This approximation must be closer than 10 nm (arrows). In the intervening space, there can be no fibrous tissue. B, In biointegration, the implant and bone are fused and continuous with one another. Osseointegration commonly occurs with titanium alloys, whereas biointegration occurs with ceramics and ceramic-coated metallic implants.
In recent years, various surface configurations have been proposed as means of improving the cohesiveness of the implant/tissue interface, maximizing load transfer, minimizing relative motion between the implant and tissue, minimizing fibrous integration and loosening, and lengthening the service life of the construct. Because of the necessity of developing a stable interface before loading, effort has been placed on developing materials and methods to accelerate tissue apposition to the implant surface. Surface-roughened implants and ceramic coatings have been implemented into clinical practice. Other, more experimental techniques include electrical stimulation, bone grafting, and the use of growth factors and other tissue engineering approaches described in Chapter 16.
The application of bioactive ceramics as implant materials was traditionally limited to their use as bone bonding and augmentation materials. There has been interest in coating titanium alloys with bioactive materials to promote an implant bone connection. Bioactive ceramic materials are more than just biocompatible. The use of the term bioactive implies that they have the ability to elicit a favorable tissue response when implanted in vivo. These ceramics form a direct chemical bond with natural tissues and are most often designed to bioresorb or biodegrade, having high solubility. Commonly implanted dental ceramics include the calcium phosphates with various calcium-to-phosphorus ratios (e.g., hydroxyapatite and tricalcium phosphate), bioactive glasses (mixtures of SiO2, CaO, P2O5, and sometimes Na2O, and MgO), and glass ceramics.
Important examples of bioactive glasses and glass ceramics include Bioglass (a glass containing a mixture of silica, phosphate, calcia, and soda); Ceravital (which has a different alkali oxide concentration compared to Bioglass); Biogran (which has a different physical conformation compared to Bioglass); and glass ceramic A-W (a glass ceramic–containing crystalline oxyapatite and fluorapatite (Ca10[PO4]6[O,F2]) and β-wollastonite (SiO2CaO) in a MgO-CaO-SiO2 glassy matrix). Additionally, many other glass and glass-ceramic compositions, based on recently developed sol-gel synthesis methods, are being developed. Calcium phosphate ceramics vary in composition, depending on processing-induced physical and chemical changes. Among this group are the apatite ceramics, and of particular interest is hydroxyapatite (HA). This is the synthetic version of the inorganic phase found in tooth and bone and is the bioactive ceramic material that has been most extensively investigated.
The impetus for using synthetic HA as a biomaterial stems from the perceived advantage of using a material similar to the mineral phase in natural tissues for replacing these materials. Because of this similarity, better tissue bonding is expected. Additional perceived advantages of HA and other bioactive ceramics include low thermal and electrical conductivity, elastic properties similar to those of bone, control of in vivo degradation rates through control of material properties, and the possibility of the ceramic functioning as a barrier to metallic corrosion products when it is coated onto a metal substrate.
However, temperature-induced phase transformations while processing HA provoke considerable changes in its in vitro dissolution behavior, and the altered structure changes the biological reaction to the material. Given the multitude of chemical compositions and structures resulting from processing bioactive ceramics and the resultant fact that pure HA is rarely used, the broader term calcium phosphate ceramics (CPCs) has been proposed in lieu of the more specific term hydroxyapatite. Each individual calcium phosphate ceramic is then defined by its own unique set of chemical and physical properties.
Although calcium phosphate ceramics are too brittle and too stiff to serve as stand-alone dental implants for prosthetic tooth replacement, there has been continuing interest in using a thin (50 to 75 μm) layer of ceramic materials to coat the surface of metallic implants. This provides the beneficial osseointegration characteristics of the ceramic combined with the high strength of the metallic alloy. Most manufacturers provide implants coated with calcium phosphate ceramic for use in sites where poor bone quality exists. A major limitation in using this concept in all clinical situations, however, has revolved around the inability to predict and maintain the bond strength of the coating to the metal. When the bioactive ceramic material resorbs in vivo, an unpredictable change occurs in the implant-bone interface, and implant micromotion and loosening may occur. This makes the long-term stability of these implants uncertain.
If successful, the ceramic coating becomes completely fused with the surrounding bone. In this case, the interface is called biointegration rather than osseointegration, and there is no intervening space between the bone and the implant (see Figure 15-2). A number of ceramic coatings have been used in this manner.
Typically, these coatings have been applied to the surface of an implant via a plasma-spray deposition process. This results in a complex mixture of HA, tricalcium phosphate, and tetracalcium phosphate in the coating, rather than a recapitulation of the starting powder mixture. Physical properties of importance to the functionality of calcium phosphate ceramics include powder particle size, particle shape, pore size, pore shape, pore-size distribution, specific surface area, phases present, crystal structure, crystal size, grain size, density, coating thickness, hardness, and surface roughness.
The long-term integrity of the ceramic coating in vivo is not known, but evidence indicates that these coatings will resorb over time. Additionally, results of ex vivo push-out tests indicate that the ceramic-metal bond fails before the ceramic-tissue bond and is the weak link in the system. Thus the weak ceramic-metal bond and the integrity of that interface over a lengthy service life of functional loading is reason for concern.
Factors Affecting the Endosteal Implant
Geometry
Two primary objectives influence a patient’s decision to pursue dental implant treatment: aesthetics and function. To fulfill these objectives over an extended period, a dental implant must be capable of withstanding the occlusal stresses generated in the oral environment and in turn transfer this load to the supporting tissues. Not only must loads be transferred, they should also be of an appropriate direction and magnitude so tissue viability is maintained. In this respect, the implant principally acts to minimize and distribute the biomechanical forces. The forces are characterized by their magnitude, duration, and type. The ability to transfer force largely depends on attaining interfacial fixation. The interface between the implant and bone must stabilize in as short a time as possible postoperatively, and once stable, must remain stable throughout its service life. Designing an “optimal” implant that meets all the foregoing objectives requires the integration of material, physical, chemical, mechanical, biological, and economic factors.
Magnitude of the Force
The amount of load applied during normal chewing varies greatly, depending on location and state of the patient’s dentition. Bite force values reported in the literature range from about 40 to 1250 N. The magnitude of force is greatest in the molar region because this area acts like the hinge of a lever (Figure 15-3). The incisor region, in comparison, experiences about 10% of the magnitude seen in the posterior segment. This difference in load borne by the teeth and supporting bone dictates differences in mechanical requirements between anterior and posterior implants. Because stress depends not only on the applied load, but also on the area over which this load is distributed, the loss of some teeth by a patient will greatly increase the stresses applied to the remaining teeth and implants in partially edentulous patients.
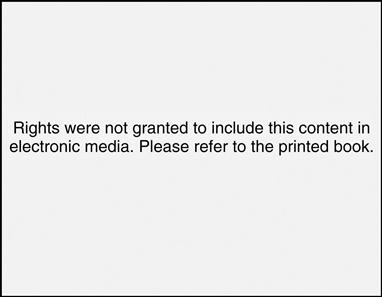
Rights were not granted to include this figure in electronic media. Please refer to the printed book. (From Rugh SD, Solberg WK: Behav. Res. Meth. Instrum. 4, 125, 1972.)
A prime requirement for any dental implant is adequate supporting bone height, width, and density. It is well established that bone grows in response to strain, and the presence of an increasing magnitude of stress applied to the bone will result in an increasing magnitude of resorption or loss of bone. However, in the absence of a critical level of strain for normal bone maintenance, the bone will also resorb. Therefore, if the patient has been edentulous for a prolonged time, the underlying bone will have resorbed and become less dense. It is common to place implants preferentially in the anterior mandible, because this region has the greatest trabecular bone density when compared with the premolar or molar regions in both dentate and edentulous patients. When planning implant treatment, careful consideration must be given to the load distribution.
A great majority of the materials considered to be biocompatible are not suitable for use as implants, because their ultimate strength is not high enough to withstand the forces to which they are subjected during normal function. But, in order to survive and continue to function effectively, it is not only the ultimate strength, but also the modulus of elasticity (or stiffness) of the material that must be considered. Unless the bone experiences at least 50 microstrain on a routine basis, it will begin to resorb. Most ceramic materials are extremely stiff, for example, polycrystalline aluminum oxide has a modulus of elasticity ≈372 GPa. This stiffness is too high to transfer an adequate amount of an applied force to the bone. Instead, the stiffer implant material will carry a disproportionate amount of the load, causing stress shielding of the bone. In contrast, titanium has a modulus of elasticity ≈100 GPa, still too high to be ideal, but much closer to that of bone (≈20 GPa). It will permit normal physiological loading of the bone.
Duration of the Force
When considering repetitive loading such as occurs during mastication, it is more appropriate to consider the endurance limit of a material rather than its ultimate strength. The endurance limit is the highest amount of stress to which a material may be repeatedly subjected without failing. This limit is typically only about half of the ultimate strength for the material.
The tooth root-form implant is designed to be loaded parallel to the long axis and is vulnerable to fatigue failure from cyclic bending loads. These bending loads often result from premature contact, bruxism, inappropriate occlusal schemes, and the use of angled abutments. Off-axis loading should be avoided in design of the implant superstructure.
Type of Force
An implant experiences three types of loads in function: tensile forces, compressive forces, and shear forces. As discussed, a well-designed implant transfers and distributes these forces to the supporting bone. Bone is composed of both inorganic and organic constituents, and the inorganic components render it strongest when loaded in compression. Bone is about 30% weaker when placed in tension, and nearly 70% weaker when subjected to shear forces. Therefore, occlusion is a crucial consideration in designing the implant loading.
Smooth-sided cylindrical implant designs place the interface between the implant and the bone in nearly pure shear, the weakest possible loading scenario. These implant designs rely either on microscopic texturing of the implant body to offer some mechanical interlocking and provide retention, or else they rely on a coating. If the coating on these fails or resorbs, bone loss usually results from the lack of load transfer.
In contrast, screw-shaped implants have threads to engage the bone in compression and transfer the applied load. The thread designs have been extensively researched to provide a minimum of shear forces and maximal compression to the bone. This allows for the most favorable bone response. A number of recent thread designs have been introduced that use rounded thread tips (to reduce shear forces at the tip), changes in the thread angle (to maximize compression), two or more thread profiles on the same implant (which will cut at different locations in the osteotomy, thereby increasing the contact area), and/or a reduced thread height accompanied by an increased thread pitch (spreading the implant loading over a greater contact area while simultaneously increasing the strength of the implant body).
The concept of osseointegration around cylindrical or screw-shaped implants represents a situation of bone ongrowth. An alternative method of implant fixation is based on bone tissue ingrowth into roughened or three-dimensional porous surface layers. Such designs incorporating sintered beads or a sintered wire mesh, are typical of orthopedic implants. Recently, implants with porous metal bodies have been developed and marketed. These new designs incorporate macroscale porosity or porous cellular structures, resembling cancellous bone. This results in a modulus of elasticity close to that of bone, thereby reducing the resulting stress shielding. Such retention systems have been shown to have higher bone/metal shear strength than other types of fixation. Increased interfacial shear strength results in a better stress transfer from the implant to the surrounding bone, a more uniform stress distribution between the implant and bone, and lower stresses in the implant. And, in principle, the r/>
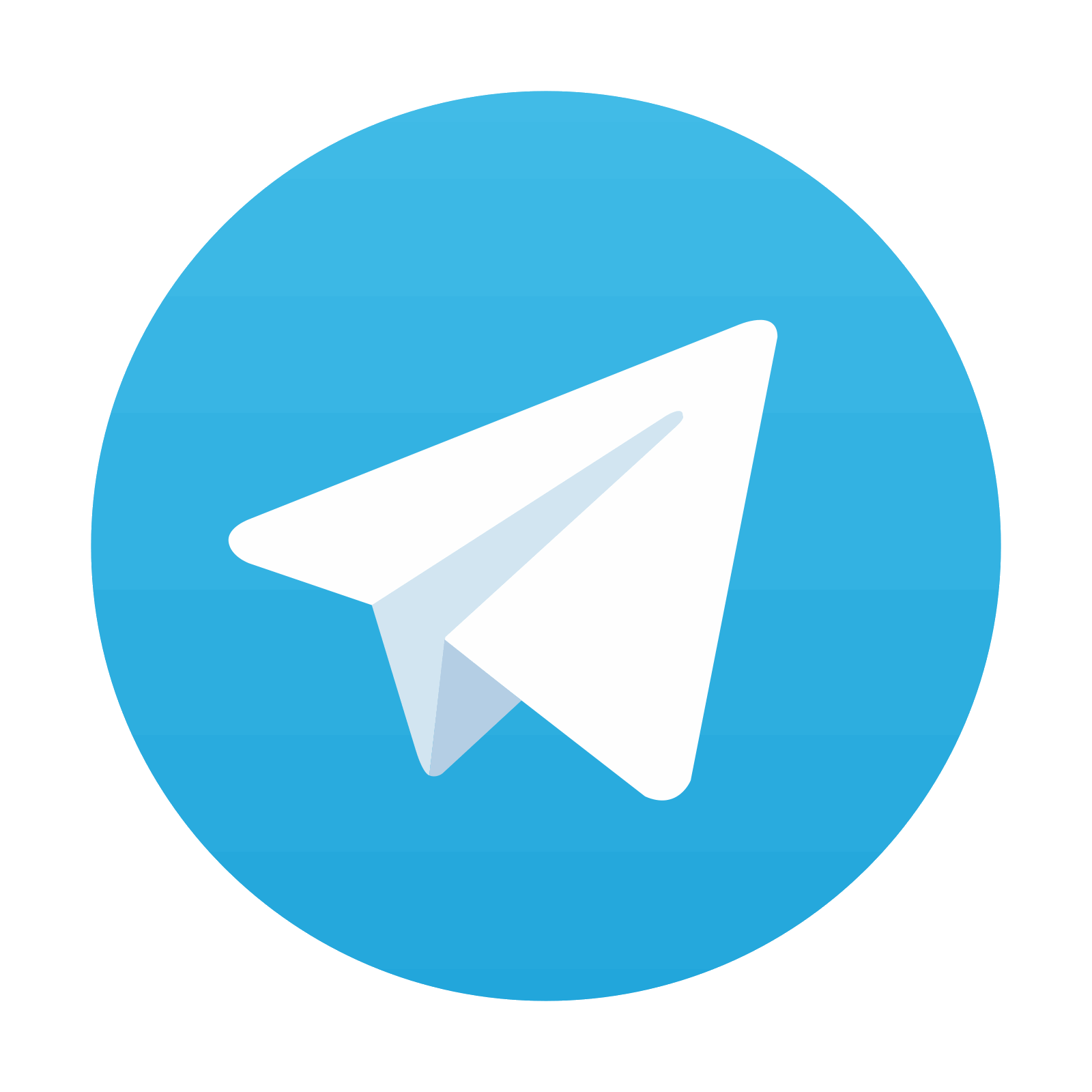
Stay updated, free dental videos. Join our Telegram channel

VIDEdental - Online dental courses
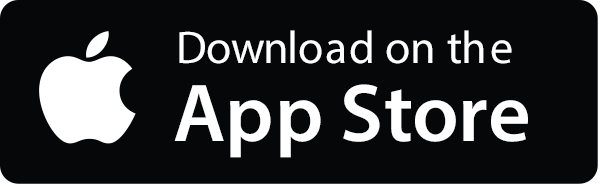
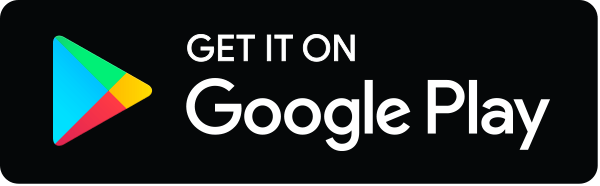