The Biologic Basis of Orthodontic Therapy
PERIODONTAL AND BONE RESPONSE TO NORMAL FUNCTION
Periodontal Ligament Structure and Function
Role of the Periodontal Ligament in Eruption and Stabilization of the Teeth
PERIODONTAL LIGAMENT AND BONE RESPONSE TO SUSTAINED FORCE
Biologic Control of Tooth Movement
Effects of Force Distribution and Types of Tooth Movement
Effects of Force Duration and Force Decay
Drug Effects on the Response to Orthodontic Force
Effects of Local Injury: Corticotomy and Accelerated Tooth Movement
In this chapter, the response of periodontal structures to orthodontic force is discussed first, and then the response of skeletal areas distant from the dentition is considered, drawing on the background of normal growth provided in Chapters 2 through 4.
Periodontal and Bone Response to Normal Function
Periodontal Ligament Structure and Function
Each tooth is attached to and separated from the adjacent alveolar bone by a heavy collagenous supporting structure, the periodontal ligament (PDL). Under normal circumstances, the PDL occupies a space approximately 0.5 mm in width around all parts of the root. By far the major component of the ligament is a network of parallel collagenous fibers, inserting into cementum of the root surface on one side and into a relatively dense bony plate, the lamina dura, on the other side. These supporting fibers run at an angle, attaching farther apically on the tooth than on the adjacent alveolar bone. This arrangement, of course, resists the displacement of the tooth expected during normal function (Figure 8-1).
The principal cellular elements in the PDL are undifferentiated mesenchymal cells and their progeny in the form of fibroblasts and osteoblasts. The collagen of the ligament is constantly being remodeled and renewed during normal function. The same cells can serve as both fibroblasts, producing new collagenous matrix materials, and fibroclasts, destroying previously produced collagen.1 Remodeling and recontouring of the bony socket and the cementum of the root is also constantly being carried out, though on a smaller scale, as a response to normal function.
Fibroblasts in the PDL have properties similar to osteoblasts, and new alveolar bone probably is formed by osteoblasts that differentiated from the local cellular population.2 Bone and cementum are removed by specialized osteoclasts and cementoclasts, respectively. These multinucleated giant cells are quite different from the osteoblasts and cementoblasts that produce bone and cementum. Despite years of investigation, their origin remains controversial. Most are of hematogenous origin; some may be derived from stem cells found in the local area.3
Response to Normal Function
The extent of bone bending during normal function of the jaws (and other skeletal elements of the body) is often not appreciated. The body of the mandible bends as the mouth is opened and closed, even without heavy masticatory loads. Upon wide opening, the distance between the mandibular molars decreases by 2 to 3 mm. In heavy function, individual teeth are slightly displaced as the bone of the alveolar process bends to allow this to occur, and bending stresses are transmitted over considerable distances. Bone bending in response to normal function generates piezoelectric currents (Figure 8-2) that appear to be an important stimulus to skeletal regeneration and repair (see further discussion later in this chapter). This is the mechanism by which bony architecture is adapted to functional demands.
Very little of the fluid within the PDL space is squeezed out during the first second of pressure application. If pressure against a tooth is maintained, however, the fluid is rapidly expressed, and the tooth displaces within the PDL space, compressing the ligament itself against adjacent bone. Not surprisingly, this hurts. Pain is normally felt after 3 to 5 seconds of heavy force application, indicating that the fluids are expressed and crushing pressure is applied against the PDL in this amount of time (Table 8-1). The resistance provided by tissue fluids allows normal mastication, with its force applications of 1 second or less, to occur without pain.
TABLE 8-1
Physiologic Response to Heavy Pressure Against a Tooth
Time (seconds) | Event |
<1 | PDL fluid incompressible, alveolar bone bends, piezoelectric signal generated |
1-2 | PDL fluid expressed, tooth moves within PDL space |
3-5 | PDL fluid squeezed out, tissues compressed; immediate pain if pressure is heavy |
Although the PDL is beautifully adapted to resist forces of short duration, it rapidly loses its adaptive capability as the tissue fluids are squeezed out of its confined area. Prolonged force, even of low magnitude, produces a different physiologic response—remodeling of the adjacent bone. Orthodontic tooth movement is made possible by the application of prolonged forces. In addition, light prolonged forces in the natural environment—forces from the lips, cheeks, or tongue resting against the teeth—have the same potential as orthodontic forces to cause the teeth to move to a different location (see the discussion of equilibrium factors in Chapter 5).
Role of the Periodontal Ligament in Eruption and Stabilization of the Teeth
The phenomenon of tooth eruption makes it plain that forces generated within the PDL itself can produce tooth movement. After a tooth emerges into the mouth, further eruption depends on metabolic events within the PDL, including but perhaps not limited to formation, cross-linkage, and maturational shortening of collagen fibers (see Chapter 3). This process continues, although at a reduced rate, into adult life. A tooth whose antagonist has been extracted will often begin to erupt again after many years of apparent quiescence.
The continuing presence of this mechanism indicates that it may produce not only eruption of the teeth under appropriate circumstances but also active stabilization of the teeth against prolonged forces of light magnitude. It is commonly observed that light prolonged pressures against the teeth are not in perfect balance, as would seem to be required if tooth movement were not to occur (Figure 8-3). The ability of the PDL to generate a force and thereby contribute to the set of forces that determine the equilibrium situation, probably explains this.
Periodontal Ligament and Bone Response to Sustained Force
Biologic Control of Tooth Movement
Before discussing in detail the response to orthodontic force, it is necessary to consider the biologic control mechanisms that lead from the stimulus of sustained force application to the response of orthodontic tooth movement. Two possible control elements, biologic electricity and pressure-tension in the PDL that affects blood flow, are contrasted in the two major theories of orthodontic tooth movement. The bioelectric theory relates tooth movement at least in part to changes in bone metabolism controlled by biologic electricity that are produced by light pressure against the teeth. The pressure–tension theory relates tooth movement to cellular changes produced by chemical messengers, traditionally thought to be generated by alterations in blood flow through the PDL. Pressure and tension within the PDL, by reducing (pressure) or increasing (tension) the diameter of blood vessels in the ligament space, could certainly alter blood flow. The two theories are neither incompatible nor mutually exclusive, and it appears that both mechanisms may play a part in the biologic control of tooth movement.4
Biologic Electricity
Piezoelectric signals have two unusual characteristics: (1) a quick decay rate (i.e., when a force is applied, a piezoelectric signal is created in response that quickly dies away to zero even though the force is maintained) and (2) the production of an equivalent signal, opposite in direction, when the force is released (see Figure 8-2).
There is also a reverse piezoelectric effect. Not only will the application of force cause distortion of crystalline structure and with it an electric signal, but also application of an electric field can cause a crystal to deform and produce force in doing so. Reverse piezoelectricity has no place in natural control systems, at least as far as is presently known, but there are intriguing possibilities for using external electric fields to promote bone healing and regeneration after injury.5
On the other hand, sustained force of the type used to induce orthodontic tooth movement does not produce prominent stress-generated signals. As long as the force is sustained, nothing happens. If stress-generated signals were important in producing the bone remodeling associated with orthodontic tooth movement, a vibrating application of pressure would be advantageous. Although earlier experiments indicated little or no advantage in vibrating over sustained force for the movement of teeth,6 this idea has been revived recently and is discussed below in the section of this chapter on possibilities for accelerating tooth movement. It still is the case, however, that the stress-generated signals that are so important for normal skeletal function have little if anything to do with the response to orthodontic tooth movement.
Electromagnetic fields also can affect cell membrane potentials and permeability and thereby trigger changes in cellular activity. In animal experiments, a pulsed electromagnetic field increased the rate of tooth movement, apparently by shortening the initial “lag phase” before tooth movement begins.7 This does not mean that the fields generated by small magnets attached to the teeth to generate tooth-moving forces (see Chapter 9) could change the basic biology of the response to force. Claims that moving teeth with magnetic force reduces pain and mobility are not supported by evidence.
Pressure–Tension in Periodontal Ligament
The pressure–tension theory, the classic theory of tooth movement, relies on chemical rather than electric signals as the stimulus for cellular differentiation and ultimately tooth movement. Chemical messengers are important in the cascade of events that lead to remodeling of alveolar bone and tooth movement, and both mechanical compression of tissues and changes in blood flow can cause their release. Because this does explain the course of events reasonably well,8 it remains the basis of the following discussion.
There is no doubt that sustained pressure against a tooth causes the tooth to shift position within the PDL space, compressing the ligament in some areas while stretching it in others. The mechanical effects on cells within the ligament cause the release of cytokines, prostaglandins, and other chemical messengers. In addition, blood flow is decreased where the PDL is compressed (Figure 8-4), while it is maintained or increased where the PDL is under tension (Figure 8-5). These alterations in blood flow also quickly create changes in the chemical environment. For instance, oxygen levels certainly would fall in the compressed area and carbon dioxide (CO2) levels would increase, while the reverse might occur on the tension side. These chemical changes, acting either directly or by stimulating the release of other biologically active agents, then would stimulate cellular differentiation and activity. In essence, this view of tooth movement shows three stages: (1) initial compression of tissues and alterations in blood flow associated with pressure within the PDL, (2) the formation and/or release of chemical messengers, and (3) activation of cells.
Effects of Force Magnitude
The heavier the sustained pressure, the greater should be the reduction in blood flow through compressed areas of the PDL, up to the point that the vessels are totally collapsed and no further blood flows (Figure 8-6). That this theoretical sequence actually occurs has been demonstrated in animal experiments, in which increasing the force against a tooth causes decreasing perfusion of the PDL on the compression side (see Figures 8-4 and 8-5).9 Let us consider the time course of events after application of orthodontic force, contrasting what happens with heavy versus light force (Table 8-2).
TABLE 8-2
Physiologic Response to Sustained Pressure Against a Tooth
PDL, Periodontal ligament; cAMP, cyclic adenosine monophosphate.
What happens in the first hours after sustained force is placed against a tooth, between the onset of pressure and tension in the PDL and the appearance of second messengers a few hours later? Experiments have shown that prostaglandin and interleukin-1 beta levels increase within the PDL within a short time after the application of pressure, and it is clear now that prostaglandin E (PgE) is an important mediator of the cellular response. Since prostaglandins are released when cells are mechanically deformed, it appears that prostaglandin release is a primary rather than a secondary response to pressure. At the molecular level, we are beginning to understand how these effects are created. Focal adhesion kinase (FAK) appears to be the mechanoreceptor in PDL cells, and its compression is at least part of the reason that PgE2 is released.10 Experiments have shown that concentrations of the receptor activator of nuclear factor-kappa ligand (RANKL) and osteoprotegerin (OPG) in gingival crevicular fluid increase during orthodontic tooth movement, which suggests that PDL cells under stress may induce the formation of osteoclasts through upregulation of RANKL.11 Other chemical messengers, particularly members of the cytokine family but also nitric oxide (NO) and other regulators of cellular activity, also are involved.12 Since drugs of various types can affect both prostaglandin levels and other potential chemical messengers, it is clear that pharmacologic modification of the response to orthodontic force is more than just a theoretical possibility (see further discussion below).
For a tooth to move, osteoclasts must be formed so that they can remove bone from the area adjacent to the compressed part of the PDL. Osteoblasts also are needed to form new bone on the tension side and remodel resorbed areas on the pressure side. Prostaglandins have the interesting property of stimulating both osteoclastic and osteoblastic activity, making it particularly suitable as a mediator of tooth movement. If parathyroid hormone is injected, osteoclasts can be induced in only a few hours, but the response is much slower when mechanical deformation of the PDL is the stimulus, and it can be up to 48 hours before the first osteoclasts appear within and adjacent to the compressed PDL. Studies of cellular kinetics indicate that they arrive in two waves, implying that some (the first wave) may be derived from a local cell population, while others (the larger second wave) are brought in from distant areas via blood flow. These cells attack the adjacent lamina dura, removing bone in the process of “frontal resorption,” and tooth movement begins soon thereafter. At the same time, but lagging somewhat behind so that the PDL space becomes enlarged, osteoblasts (recruited locally from progenitor cells in the PDL) form bone on the tension side and begin remodeling activity on the pressure side.13
Because of its histologic appearance as the cells disappear, an avascular area in the PDL traditionally has been referred to as hyalinized (see Figure 8-4). Despite the name, the process has nothing to do with the formation of hyaline connective tissue. It represents the inevitable loss of all cells when the blood supply is totally cut off. When this happens, remodeling of bone bordering the necrotic area of the PDL must be accomplished by cells derived from adjacent undamaged areas.
After a delay of several days, cellular elements begin to invade the necrotic (hyalinized) area. More importantly, osteoclasts appear within the adjacent bone marrow spaces and begin an attack on the underside of the bone immediately adjacent to the necrotic PDL area (Figure 8-7). This process is appropriately described as undermining resorption, since the attack is from the underside of the lamina dura. When hyalinization and undermining resorption occur, an inevitable delay in tooth movement results. This is caused first by a delay in stimulating differentiation of cells within the marrow spaces, and second because a considerable thickness of bone must be removed from the underside before any tooth movement can take place. The different time course of tooth movement when frontal resorption is compared with undermining resorption is shown graphically in Figure 8-8.
Not only is tooth movement more efficient when areas of PDL necrosis are avoided, but pain is also lessened. However, even with light forces, small avascular areas are likely to develop in the PDL, and tooth movement will be delayed until these can be removed by undermining resorption. The smooth progression of tooth movement with light force shown in Figure 8-8 may be an unattainable ideal when continuous force is used. In clinical practice, tooth movement usually proceeds in a more stepwise fashion because of the inevitable areas of undermining resorption.
Effects of Force Distribution and Types of Tooth Movement
The simplest form of orthodontic movement is tipping. Tipping movements are produced when a single force (e.g., a spring extending from a removable appliance) is applied against the crown of a tooth. When this is done, the tooth rotates around its “center of resistance,” a point located about halfway down the root. (A further discussion of the center of resistance and its control follows in Chapter 9.) When the tooth rotates in this fashion, the PDL is compressed near the root apex on the same side as the spring and at the crest of the alveolar bone on the opposite side from the spring (Figure 8-9). Maximum pressure in the PDL is created at the alveolar crest and at the root apex. Progressively less pressure is created as the center of resistance is approached, and there is minimum pressure at that point.
In tipping, only one-half of the PDL area that could be loaded actually is. As shown in Figure 8-9, the “loading diagram” consists of two triangles, covering half of the total PDL area. On the other hand, pressure in the two areas where it is concentrated is high in relation to the force applied to the crown. For this reason, forces used to tip teeth must be kept quite low. Both experiments with animals and clinical experience with humans suggest that tipping forces for a single-rooted tooth should not exceed approximately 50 gm, and lighter forces are better for smaller teeth (which have a smaller PDL).
If two forces are applied simultaneously to the crown of a tooth, the tooth can be moved bodily (translated), that is, the root apex and crown move in the same direction the same amount. In this case, the total PDL area is loaded uniformly (Figure 8-10). It is apparent that to produce the same pressure in the PDL and therefore the same biologic response, twice as much force would be required for bodily movement as for tipping. To move a tooth so that it is partially tipped and partially translated would require forces intermediate between those needed for pure tipping and bodily movement (Table 8-3).
TABLE 8-3
Optimum Forces for Orthodontic Tooth Movement
Type of movement | Force* (gm) |
Tipping | 35-60 |
Bodily movement (translation) | 70-120 |
Root uprighting | 50-100 |
Rotation | 35-60 |
Extrusion | 35-60 |
Intrusion | 10-20 |
*Values depend in part on the size of the tooth; smaller values appropriate for incisors, higher values for multirooted posterior teeth.
For many years, it was considered essentially impossible to produce orthodontic intrusion of teeth. Now it is clear that clinically successful intrusion can be accomplished, but only if very light forces are applied to the teeth. Light force is required for intrusion because the force will be concentrated in a small area at the tooth apex (Figure 8-11). As with extrusion, the tooth probably will tip somewhat as it is intruded, but the force still will be concentrated at the apex. Only if the force is kept very light can intrusion be expected.
Effects of Force Duration and Force Decay
Clinical experience suggests that there is a threshold for force duration in humans in the 4 to 8 hour range and that increasingly effective tooth movement is produced if force is maintained for longer durations. Although no firm experimental data are available, a plot of efficiency of tooth movement as a function of force duration would probably look like Figure 8-12. Continuous forces, produced by fixed appliances that are not affected by what the patient does, produce more tooth movement than removable appliances unless the removable appliance is present almost all the time. Removable appliances worn for decreasing fractions of time produce decreasing amounts of tooth movement.
Duration of force has another aspect, related to how force magnitude changes as the tooth responds by moving. Only in theory is it possible to make a perfect spring, one that would deliver the same force day after day, no matter how much or how little the tooth moved in response to that force. In reality, some decline in force magnitude (i.e., force decay) is noted with even the springiest device after the tooth has moved a short distance (though with the superelastic nickel–titanium materials discussed in Chapter 10, the decrease is amazingly small). With many orthodontic devices, the force may drop all the way to zero. From this perspective, orthodontic force duration is classified (Figure 8-13) by the rate of decay as:
• Continuous—force maintained at some appreciable fraction of the original from one patient visit to the next
• Interrupted—force levels decline to zero between activations
• Intermittent—force levels decline abruptly to zero intermittently, when an orthodontic appliance or elastic attached to a fixed appliance is removed by the patient, and then return to the original level some time later. When tooth movement occurs, force levels will decrease as they would with a fixed appliance (i.e., the intermittent force can also become interrupted between adjustments of the appliance).
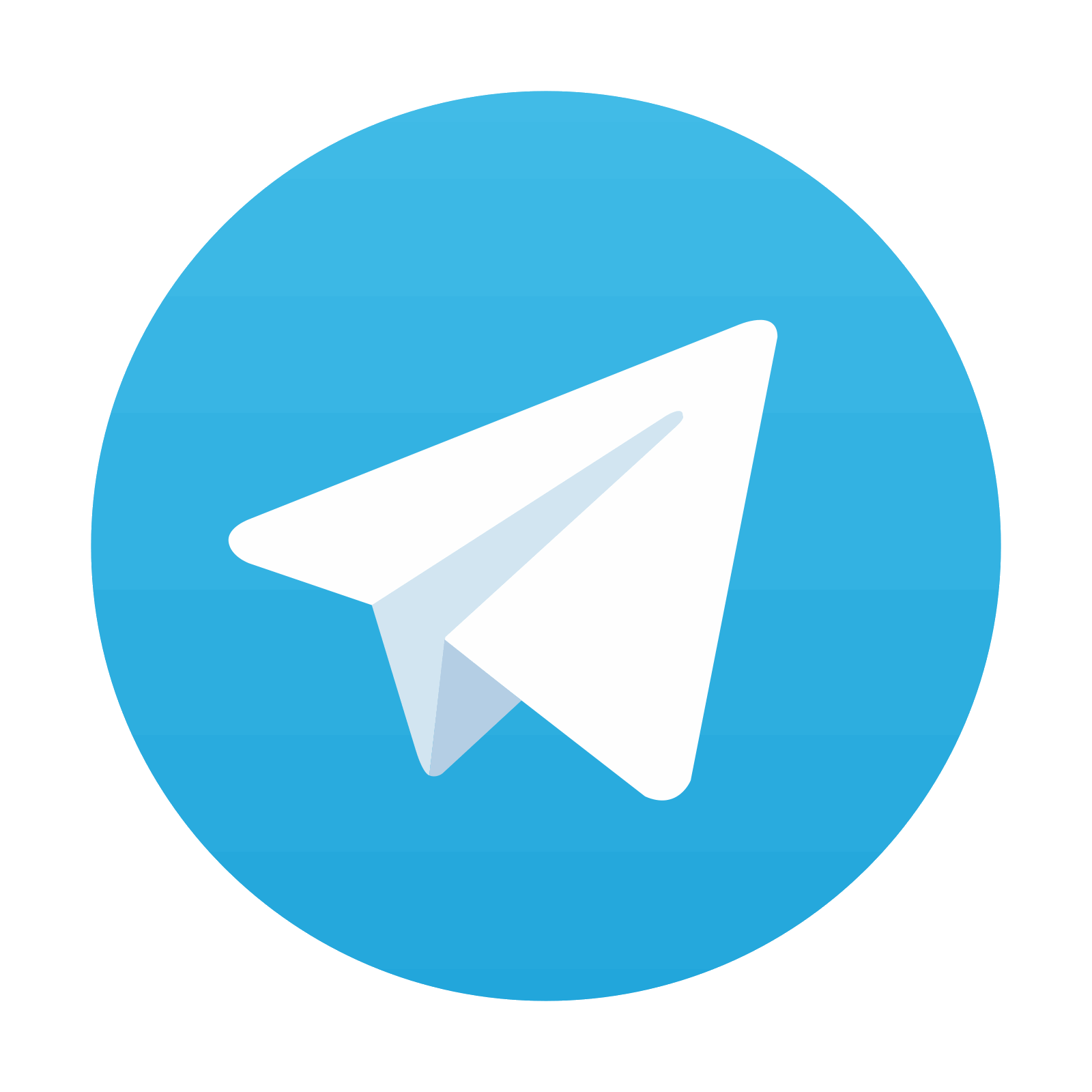
Stay updated, free dental videos. Join our Telegram channel

VIDEdental - Online dental courses
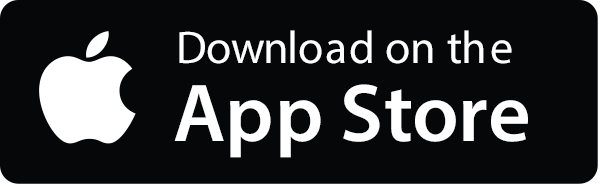
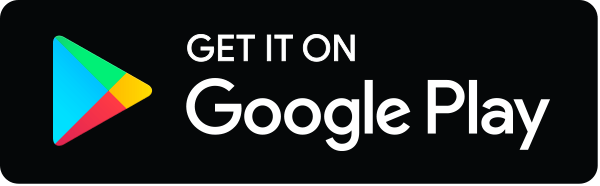