6
Tissue Reaction
Orthopaedic effects
The Herbst appliance
Forward posturing
Palatal expansion
Orthodontic effects in adult patients
Tissue reaction to orthodontic loading
Orthodontist-related factors
Patient-related factors
Orthodontically induced apical root resorption
References
Orthopaedic Effects
The treatment principles applicable to adult patients are basically limited to tooth movements and orthognathic surgery. However, some orthopaedic effects have been evidenced clinically even in adult patients when using Herbst appliances and/or forward posturing.
The Herbst Appliance
Condylar changes and modelling of the glenoid fossa following Herbst treatment have been demonstrated in both young and adult non-human primates (Woodside et al. 1987; Voudouris et al. 2003a, b). Although the hyperactivity of the lateral pterygoid muscle does not correlate to growth modifications, the reciprocal stretch of the ligament connecting the condyle to the fossa may play a role in new bone formation.
Minor skeletal discrepancies in young adults can be corrected with Herbst appliance treatment. Paulsen and Karle (2000) treated two 20-year-old patients with Class II malocclusions using the Herbst appliance and found new bone formation at the condyles. Distocranial bone apposition was visible both on the orthopantomograms and by means of computer tomography (CT). This is consistent with Ruf and Pancherz’ (1999) findings, which demonstrated that efficiency of the Herbst appliance in the correction of Class II malocclusions was maximum after the pubertal growth spurt. Evidence of modelling of the temporomandibular joints (TMJs), the condyles as well as the articular fossae in young adults was confirmed by magnetic resonance and three-dimensional X-rays (Fig. 6.1). According to Ruf and Pancherz (1999), Herbst treatment could be a possible alternative to orthognathic surgery in borderline adult skeletal Class II cases.
Fig. 6.1 (1-3) Magnetic resonance image of the temporomandibular joint tissue after Herbst appliance treatment in an adult patient. Note the tissue modelling at the condylar and fossa levels.
(Courtesy of Professor S Ruf.)
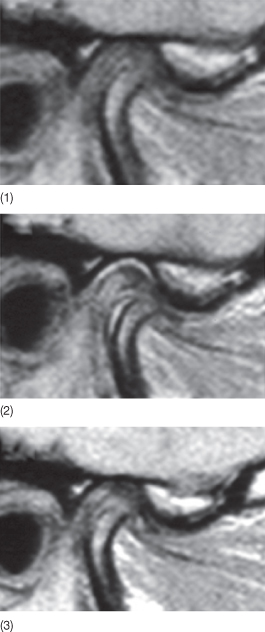
Forward Posturing
The capacity for the TMJ to model even in adult individuals is confirmed by the successful clinical results of forward posturing therapy suggested by Korn (personal communication, 2006; see Case 1 below). He demonstrated that mandibular forward posturing could be considered an alternative to surgery in patients declining surgery. Coordinating the arches so that they fit together when the mandible is postured forward resulted in a dual bite at first but, at the 5-year follow-up, the patient’s mandible could no longer be forced back into the retruded contact position. However, the precise tissue reactions at the condyles and the articular fossae still remain to be elucidated.
Case 1: Adult Patient Before and After Forward Posturing of the Mandible (Courtesy of Dr M Korn)
A 47-year-old woman with a Class II division 1 malocclusion, mutilated dentition with loss of all second molars, and chronic temporomandibular disorder presented with unsatisfactory veneers that had been constructed to correct the attritions and mask the existing crowding without orthodontics. The patient did not want to have orthognathic surgery and was given a bite plane for 11 months while she participated in a forward posture training programme. Intra-arch orthodontic tooth movement was carried out as if she was to have orthognathic surgery, but instead of orthognathic surgery, the patient trained her mandible to posture forward in a dual bite Class I relationship. At the end of treatment, she was unable to move the mandible posteriorly into its original position. Eight years following treatment, the Class I relationship was maintained and the patient had no dual bite.
Case 6.1 (1–8) Pre-treatment status. (9–16) Intra-arch levelling and alignment using a combination of fixed orthodontic appliances and a bite plane. (17–23) End of treatment status. A dual bite has been established. (24–28) Five years following treatment. The mandible can no longer be forced back into a Class II relationship.
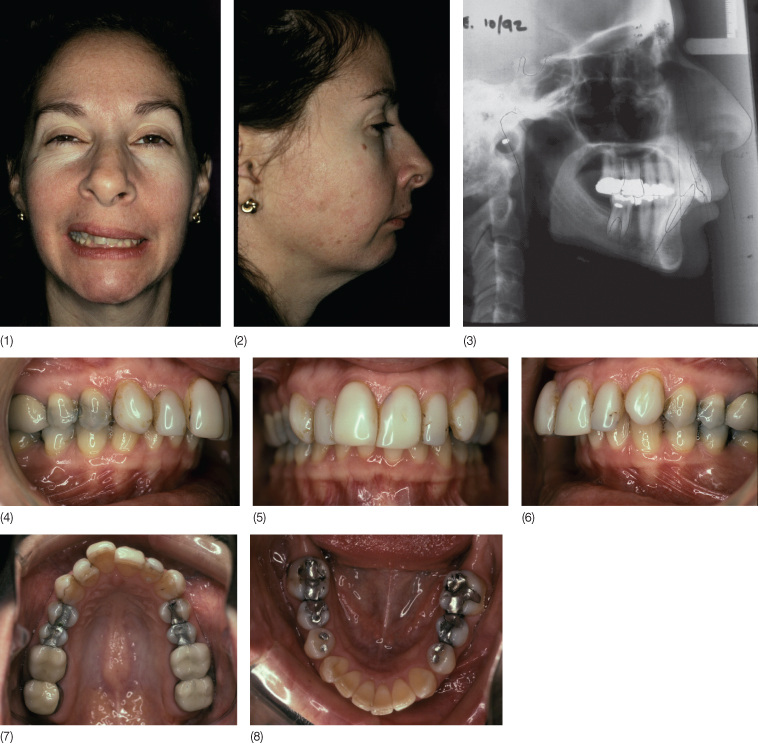
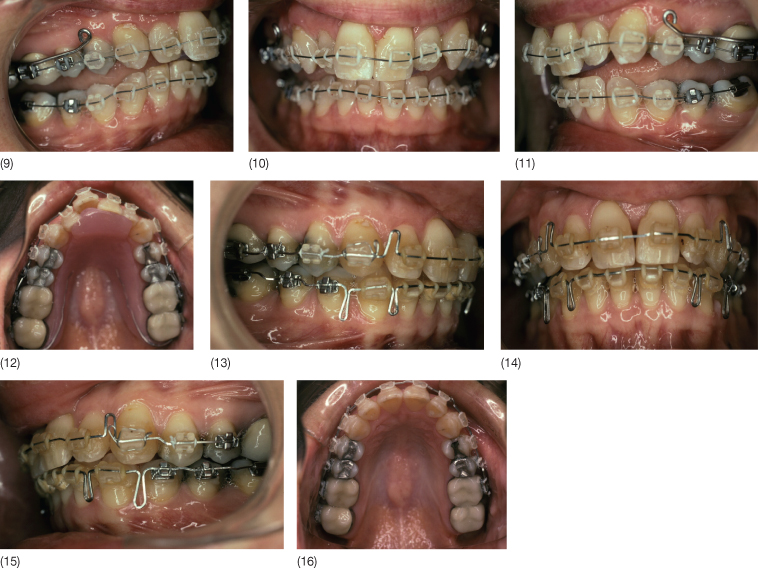
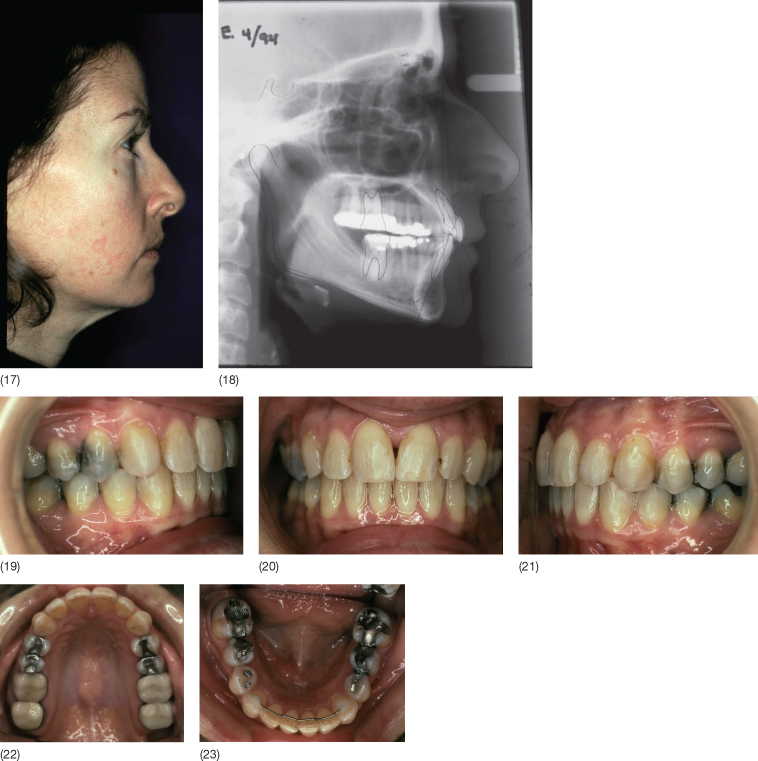
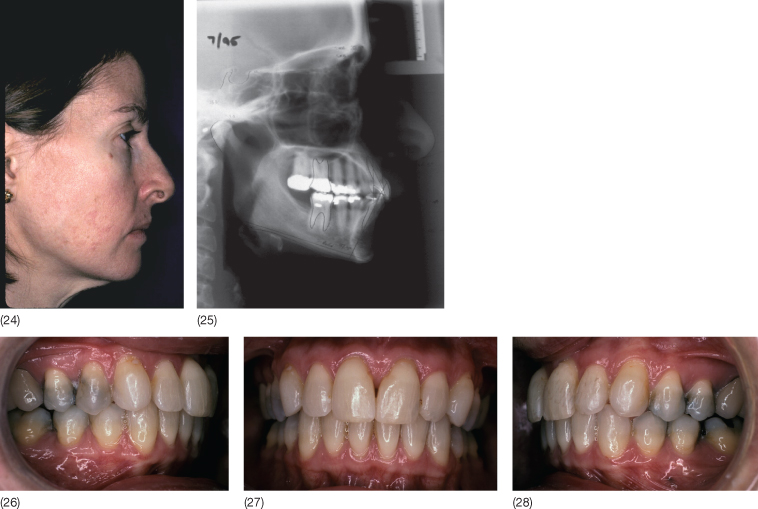
Palatal Expansion
The dental arches can be expanded by opening the midpalatal suture or by modelling of the alveolar processes. With the establishment of the occlusion of the permanent teeth, the midpalatal suture shows tight bony interdigitation and expansion here becomes difficult (Melsen 1975; Persson and Thilander 1977; Knaup et al. 2004) (Fig. 6.2).
Fig. 6.2 Histological section of the palatal suture in two 13-year-old girls. (1 and 3) Suture before expansion, material obtained at autopsy. (2 and 4) Suture 6 weeks following rapid expansion. Note the fractures occurring during expansion. Osteoclast activity is seen in the fracture areas (red arrows), together with osteoblastic activity (light blue arrows) as a sign of bone healing.
(From Melsen B (1972) A histological study of the influence of sutural morphology and skeletal maturation on rapid palatal expansion in children. Trans Eur Orthod Soc 499–507. Reproduced with kind permission of the European Orthodontics Society.)
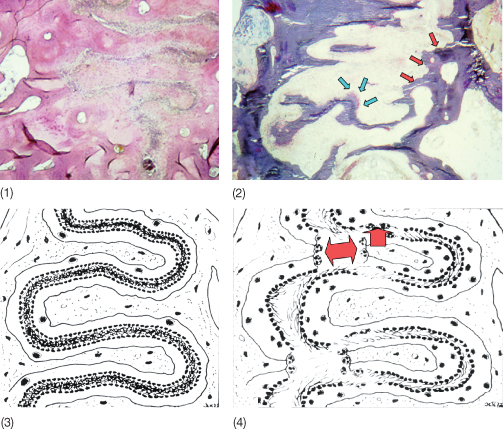
Rapid palatal expansion is performed with a cemented appliance and the skeletal contribution to the treatment result depends on the degree of opening of the suture. A histological study carried out on biopsies of the midpalatal suture following rapid palatal expansion (Hyrax) demonstrated that in children in the mixed dentition the suture opened. The increase in number of osteoblasts and osteoid formation indicated stimulation of growth, which then allowed establishment of the correct transverse relationship. Once tight interdigitation is established, rapid palatal expansion is achieved by fracture within or adjacent to the suture (Melsen 1972). As a consequence of the difficulty in widening of the suture, surgical-assisted rapid maxillary expansion (SARME) was introduced. By facilitating the separation of the two maxillary halves, the load on the posterior teeth and thereby the risk of creating buccal dehiscences is reduced. Tissue reaction at the suture following SARME or following the fracture resulting from the activation of the rapid expansion screw is comparable with that in distraction osteogenesis.
Depending on the magnitude of expansion, haemorrhage in the suture will occur. According to Chang, the vascular invasion by the blood clot is crucial for the new bone formation, due to the major osteogenic role of the paravascular cells (Chang et al. 1997). A recent study confirmed the role of the blood clot in healing, by showing that thrombin-related peptide accelerated the regeneration of bone following distraction osteogenesis (Amir et al. 2007). However, the stressed periosteum also plays a role in the healing process as tensile strain initiates the differentiation of the periosteal cells into osteogenic cells (Kanno et al. 2005). Although the risk of buccal dehiscence is reduced by using the SARME, the potential risk of periodontal damage between the upper central incisors should be taken into consideration (Cureton and Cuenin 1999).
Orthodontic Effects in Adult Patients
Tissue Reaction to Orthodontic Loading
The tissue reaction to the application of an orthodontic force is the result of the interaction between the mechanical perturbation generated by the orthodontic appliance and the modelling and remodelling of the alveolar bone. The clinician can control the force system delivered by the appliance. The tissues that are affected by the mechanical loading comprise: the root surface, the periodontal ligament (PDL) and the alveolar bone. Each tissue has its own cellular and extracellular elements and mechanical properties, and behaviour is controlled by both local and systemic factors (Verna et al. 1999).
Orthodontic tooth movement is a particular model of mechanical loading, due to the fact that the applied force to the teeth is transmitted to the alveolar bone via the PDL, whose mechanical properties are complicated by its non-isotropic structure. The reaction of the alveolar wall leading to tooth displacement has typically been ascribed as secondary to the reaction of the PDL. However, tooth movement depends on the turnover of the alveolar bone surrounding the PDL and this interaction is still a matter of debate in the field of orthodontic research. We still do not know all steps in the cascade of events that transform a mechanical perturbation into a biological reaction. Based on cellular research, the traditional discussion about pressure and tension has become irrelevant, as cells can hardly discriminate between pressure and tension, and instead react to difference in strain (Ehrlich and Lanyon 2002; Benjamin and Hillen 2003).
When treating adult patients, special attention should be paid to physiological age-related changes (Barnett and Rowe 1986; Jager 1996; Krall et al. 1997; Johnson et al. 2002; Jonasson 2005; Misawa-Kageyama et al. 2007) as well as changes related to metabolic diseases and chronic medication, both being more frequent in adult patients (Johnson et al. 2002; Verna et al. 2003; Kalia et al. 2004; Verna et al. 2006). In addition to the variation related to age, the response to identical stimuli varies considerably between individuals. Experiments carried out on dogs have clearly confirmed this clinical observation (Pilon et al. 1996; von Bohl et al. 2004a,b).
The Classical Pressure–Tension Model
Within the classical view of the tissue reaction to orthodontic force systems, a clear distinction has been made between pressure and tension zones (Reitan and Rygh 1994). On the application of a force, within minutes, the tooth will be displaced inside the PDL with compression of the tissues in the direction of the tooth movement. On the tension side, widening of the PDL occurs and, within hours, the diameter of the blood vessels increases.
Based on electron microscopic studies, an outflow of blood constituents occurs in areas where the pressure leads to obstruction of blood vessels. Fibroblasts exhibited swelling of endoplasmic reticulum followed by rupture of the cytoplasmic membrane and loss of cytoplasm (Rygh and Brudvik 1995). Collagen fibrils in the PDL, nevertheless, retain their banded appearance but the glassy appearance of these areas gave this process the name hyalinization, which is in fact a sterile necrosis caused by the ischaemia. The hyalinized zone is removed by macrophages without a ruffled border. The tooth will not move until the bone covered by hyalinized PDL is resorbed by osteoclasts recruited from the bone marrow spaces of the spongiosa (or from the subcortical marrow if no spongiosa is present; Fig. 6.3) (Baron 1975a). Once the undermining or indirect resorption has reached the alveolar wall, the tooth will become loose, and resorption in the direction of the tooth movement can continue – in the case of a light force – as frontal or direct resorption, with osteoclasts acting directly on the alveolar wall. If, however, the force level is still high enough to cause local ischaemia, renewed hyalinization and indirect resorption from the marrow spaces may occur (Fig. 6.4). Following the movement of the tooth, bone apposition is seen on the tension side (Fig. 6.5).
Fig. 6.3 Tooth movement through bone. When hyalinization occurs (1), the tooth does not move until the hyalinized tissue is removed via indirect resorption (2). In areas where no hyalinization occurs, bone is resorbed by osteoclasts recruited from the periodontal ligament, via direct resorption.
(With permission from Verna C (2000) Biology of dental movement. In Melsen B and Fiorelli G (eds) Biomechanics in Orthodontics, A Multimedia Textbook, pp. 38–67. Arezzo, Italy: Libra Ortodonzia.)
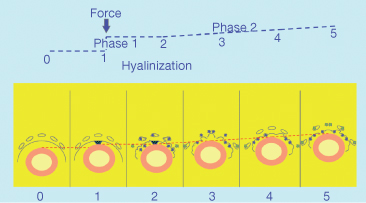
Fig. 6.4 Histological transverse section of the first root of a rat upper molar after 21 days of orthodontic force application. When the periodontal ligament is overcompressed, hyalinization occurs (red arrow) and the bone adjacent to the hyalinized tissue is resorbed by osteoclasts recruited from the marrow spaces (green arrow). The width of the periodontal ligament is not as wide as in the areas where bone resorption is carried out by the periodontal ligament cells (yellow arrow).
(With permission from Verna C (2000) Biology of dental movement. In Melsen B and Fiorelli G (eds) Biomechanics in Orthodontics, A Multimedia Textbook, pp. 38–67. Arezzo, Italy: Libra Ortodonzia.)
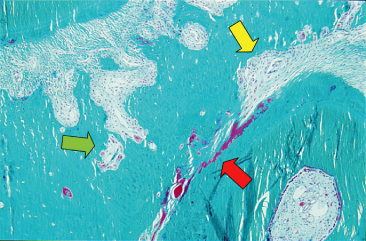
Fig. 6.5 Bone formation markers (tetracycline in yellow and calcein in green) observed in alveolar bone of a rat after the application of an orthodontic load. Note the lack of bone markers on the scalloped bone surface, typical of bone resorption in the direction of the force (right side). On the other side, a wide strip of bone markers, typical of new bone formation is seen.
(With permission from Verna C (2000) Biology of dental movement. In Melsen B and Fiorelli G (eds) Biomechanics in Orthodontics, A Multimedia Textbook, pp. 38–67. Arezzo, Italy: Libra Ortodonzia.)
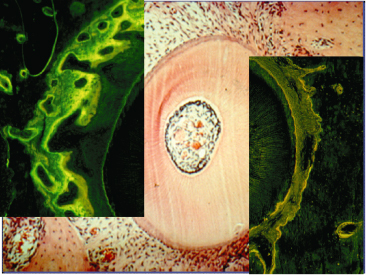
The so-called pressure–tension theory has been considered the state of the art and numerous studies have adopted this approach (Fig. 6.6). However, the notion of changes in the pressure within the PDL has also been questioned. Based on the physical properties of the PDL, that is, since it acts as a continuous hydrostatic system, Bien (1966) claimed that it is physically impossible to generate areas of specific pressure or tension within the PDL. Consequently, the initial distribution of the forces within the PDL is not constant and changes cannot be maintained . This view has been supported by Isaacson et al. (1993). Nanda and Heller (1979) have also questioned the determinant role of the PDL, showing that a biophysical modification of the PDL before tooth movement did not change the tissue reaction of the alveolar wall. They concluded that the distortion of the bone might be the primary stimulus for the cascade of events leading to the modelling of the alveolus.
Fig. 6.6 Initial tooth movement following the application of the force in the direction indicated by the arrow. In the direction of the force, the periodontal ligament (PDL) becomes initially narrow and according to the distribution of the stresses, direct or indirect resorption occurs. This area has always been termed as the pressure area. On the opposite side, widening of the PDL is observed and cellular activity is characterized by bone apposition. This area has traditionally been termed as the tension side
(With permission from Verna C (2000) Biology of dental movement. In Melsen B and Fiorelli G (eds) Biomechanics in Orthodontics, A Multimedia Textbook, pp. 38–67. Arezzo, Italy: Libra Ortodonzia.)
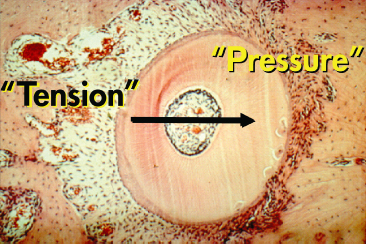
No clear consensus has been reached concerning the relative contribution of the displacement of the tooth within the PDL and of bone bending to the initial tooth movement. Some authors ascribe a substantial role in initial tooth movement to bone bending (Roberts et al. 1992). The role played by bone bending may be proportional to the initially applied force (Grimm 1972). This notion was in contradiction to Storey (1973), who studied rodents and concluded that bone bending is substantially irrelevant, being even smaller than the threshold of 0.1 mm detectable by the instruments. The methodological problem related to the differentiation between displacement within the PDL and bone bending makes it difficult to draw substantive conclusions. In spite of the different approaches and problems noted, the framework of pressure and tension areas is still a dominant theory.
A different approach to the analysis of the tissue reactions would be to start from the mechanical load, and follow the cascade occurring in the two main compartments (the PDL and the alveolar bone) separately until the same final result has been reached, i.e. modelling/remodelling of the supporting tissues. The PDL has traditionally been considered the tissue from which resorption and formation of bone were orchestrated. This is surprising since it is well known that the principal detectors of mechanical strains in bone are the osteocytes.
Periodontal Ligament
Intermittent occlusal forces resulting in tension of the periodontal fibres are necessary for the maintenance of the alveolar bone. When these occlusal forces are absent, the density of the alveolar bone is dramatically reduced (see Chapter 3, Fig. 3.21) (Picton 1964).
Loadings with a different direction or duration than those occurring during normal function result in a permanent displacement of the tooth within the viscoelastic PDL. The change in the stress/strain distribution leads to a change in the fluid distribution and, due to the oblique alveolar surface, a horizontal force will result in a shearing, leading to extrusion. This effect has been referred to as the cone effect and depends on the inclination of the alveolar wall. It is usually resisted by the supracrestal fibres, but when the PDL is compromised, extrusion may occur. In adult patients with marginal bone loss, this effect will be more pronounced, and the applied force should have a small intrusive component to avoid extrusion (Fig. 6.7).
Fig. 6.7 The cone effect. When force is applied to an inclined plane there is resolution of the force into horizontal and vertical components. The vertical force is usually resisted by the supracrestal fibres, but when the periodontal ligament is compromised, extrusion is possible.
(With permission from Verna C (2000) Biology of dental movement. In Melsen B and Fiorelli G (eds) Biomechanics in Orthodontics, A Multimedia Textbook, pp. 38–67. Arezzo, Italy: Libra Ortodonzia.)

When an orthodontic load is applied the periodontal fibres, the PDL cells, and the extracellular matrix (ECM) are submitted to a deformation and a reduction in mechanical strength, particularly on the compression side (Fukui 1993). These changes are also present on adjacent teeth (Ki 1990) but are rapidly restored with retention (Fukui et al. 2003).
In vitro studies have shown that cell deformation is related to metabolic activity (Chicurel et al. 1998). The cytoskeleton represents a possible transducer of mechanical loading by means of the interaction with the ECM via the binding glycoprotein integrins (Talic et al. 2004). It has been demonstrated that cells use tensegrity architecture for their organization, that is, cells are hard-wired to respond immediately to mechanical stresses transmitted through cell surface receptors (Fig. 6.8). The cytoskeleton is physically coupled to the extracellular matrix thus tuning cellular response at a gene regulation level (Ingber 1997). From this perspective, the integrity of the cell membrane in the mechanotransduction pathway is taken into consideration, since it has been demonstrated that trauma facilitates mechanosensitivity (Wan et al. 1999), and PDL cells have been found to experience membrane disruption and resealing on application of orthodontic force (Orellana-Lezcano et al. 2005).
Fig. 6.8 Schematization of the architectural organization of cells following tensegrity. Secondary to mechanical perturbation, the extracellular matrix (ECM) is deformed along with the cell surface (top panel). Cells adhere to the ECM via integrin (middle panel), proteins that connect the ECM with the internal cytoskeleton (bottom panel). The cytoskeleton that supports the cell architecture is thus modified and transmits the signal at the nuclear level.
(Modified from Ingber DE (1998) The architecture of life. Sci Am 278, 48–57, with permission from Scientific American.)
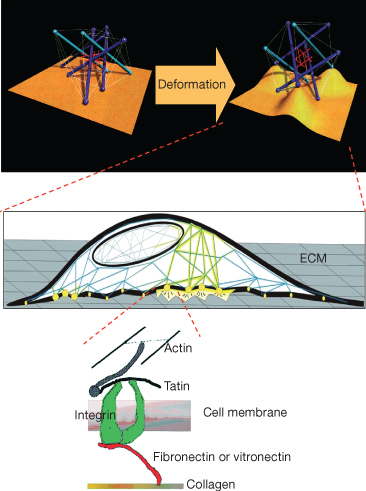
Displacement of the fluid within the PDL generates shear stresses and contributes to membrane deformation (Bien 1966). The dynamic of fluids in the PDL is mainly sustained by the vascular system that provides the PDL with essential cells and oxygen necessary for its remodelling (Rygh et al. 1986). Fifty percent of the PDL volume is occupied by blood vessels, with the highest percentage adjacent to the bony side. In the area of pressure, partial or complete occlusion of vessels leading to hyalinization is observed after 24 hours, whereas on the tension side, blood vessels are dilated. After 7 days of treatment, in the area of pressure, vascularization is re-established by invasion of vascular structures into the hyalinized areas whereas on the tension side, increased blood supply is still present (Khouw and Goldhaber 1970). The changes in density and distribution of blood vessels during tooth movement have been shown to be related to changes in the neural response (Vandevska-Radunovic et al. 1997).
The cell pool assigned to anabolic activities includes PDL fibroblasts, osteoblasts and cementoblasts. The osteoprogenitor cells reside in the environment of the blood vessels in the area of the PDL close to the alveolar wall. Pavlin and Gluhak-Heinrich (2001) concluded that the induction of differentiation and increase in cell function are the primary responses to osteogenic loading, and suggested that the mechanical signal may be targeting osteoblast precursors without the initial proliferative response. The final result is an increased production of collagen, increased ECM synthesis, up-regulation of anti-apoptotic factors and increased matrix metalloproteinase 8 (MMP-8) production, as detected in crevicular fluid (Danciu et al. 2004; Ingman et al. 2005). Catabolic activity occurs in osteoclasts originating from the hemopoietic system by the differentiation of the monocyte series. The first resorbing cells on the alveolar wall are osteoclasts, the origin of which has been attributed to post-mitotic fusion of pre-osteoclasts from the cell pool present in the PDL (Tsay et al. 1999). According to Rody et al. (2001), the clasts originated from the bone marrow, where a significant increase in proliferation is already evident on the first day of loading (Fig. 6.9).
Fig. 6.9 Paraocclusal section of a rat root after 21 days of orthodontic force application. Both direct and indirect resorption can be present on the pressure side, depending on the local stress–strain distribution of the forces.
(With permission from Verna C (2000) Biology of dental movement. In Melsen B and Fiorelli G (eds) Biomechanics in Orthodontics, A Multimedia Textbook, pp. 38–67. Arezzo, Italy: Libra Ortodonzia.)
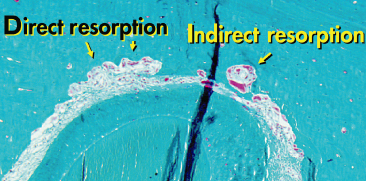
Biochemically, the mechanical perturbation causes an up-regulation of prostaglandins, release of nitric oxide (NO), and regulation of the expression of endothelial nitric oxide synthase (ecNOS). Both NO and prostaglandins are assumed to work as second messengers in the transduction pathway (Burger and Klein-Nulen 1999). The release of substance P by the sensory nerve endings in the PDL enhances prostaglandins release, leucocyte migration and secretion of lymphokines and the subsequent significant rise in levels of intracellular second messengers (cAMP, cGMP) (Davidovitch et al. 1988; Yamashiro et al. 2000). Together with prostaglandins, leukotrienes are mediators of orthodontic tooth movement, stimulating bone resorption and promoting the production of collagenase by fibroblasts that invade the hyalinized zones. This phenomenon together with a stimulation of movement of calcium in the cell has been suggested to be the mechanism by which leukotrienes enhance the remodelling of the PDL (Mohammed et al. 1989). Cytokines, soluble low-weight molecules secreted by most nucleated cells, modulate bone remodelling, coordinating metabolic responses from neighbouring cells. Among the cytokines, those that can influence connective tissue remodelling include interleukins (ILs), tumour necrosis factor (TNF) and its ligand family, interferons (IFNs), polypeptide growth factors (PGF) and colony stimulating factors (CSF).
Bone
When teeth are submitted to a force the surrounding alveolar bone undergoes deformation which is inversely proportional to the distance from the tooth apex (Asundi and Kishen 2000). Due to the stiff nature of intact in vivo bone, mechanical loads are translated to strains in the order of 0.2% (Burr et al. 1996).
Two types of activity in bone occur throughout life, modelling and remodelling. Adding to the confusion, orthodontists have applied only the word remodelling to cover both phenomena. Modelling is the dominant biological activity during growth, where it leads to changes in bone size and shape. Modelling is largely controlled by genetic programming and also by functional loads, and less so by systemic metabolic stimuli. In adult life, modelling causes the physiological drift of the alveolar socket and induces structural adaptation of bone to different mechanical demands (Frost 1990a). During tooth displacement the PDL width and bone thickness is maintained through coordinated resorption and apposition, thus resulting in drift of the whole alveolus (Baron 1975b) (Fig. 6.10). From animal studies, however, it seems that the process is more complex (Vignery and Baron 1980; Tran Van et al. 1982; Baron et al. 1984; King et al. 1992; Keeling et al. 1993; Melsen 1999). Bone formation has also been demonstrated in the direction of the force and bone resorption on the so-called tension side (Mohri et al. 1991; King et al. 1992; Zaffe and Verna 1995) . The presence of markers of both resorption and formation activities throughout the whole alveolar bone is well in agreement with the interaction between osteoblasts and osteoclasts seen in relation to the remodelling process (Fig. 6.11).
Fig. 6.10 Alveolar bone modelling during orthodontic tooth movement. After the application of 25 g of force a mesial tipping is produced in a rat first molar (1). After 14 days of treatment, the microradiographic analysis shows new bone formation ahead of the tooth on the treated side (2) compared with the untreated side (3). On the buccal side, new periosteal bone formation is observed, as shown by the wide strips of bone markers (4). Green = calcein, yellow = tetracycline. (5) Note the thin lines of bone markers on the untreated buccal side in contrast to the treated side. (6) Treated side at a higher magnification with toluidine-blue staining reveals intense new bone formation by active osteoblasts. (7) The untreated side does not show any new bone formation.
(With permission from Verna C (2000) Biology of dental movement. In Melsen B and Fiorelli G (eds) Biomechanics in Orthodontics, A Multimedia textbook, pp. 38–67. Arezzo, Italy: Libra Ortodonzia.)
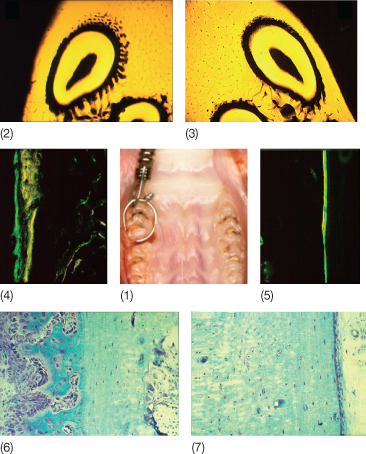
Fig. 6.11 Alveolar bone on the buccal side of a rat tooth after 14 days of orthodontic treatment. On the side facing the periodontal ligament (arrows) staining is positive for both alkaline phosphatase, i.e. bone formation (dark blue, left panel), and acid phosphatase, i.e. bone resorption (red, right panel).
(With permission from Verna C (2000) Biology of dental movement. In Melsen B and Fiorelli G (eds) Biomechanics in Orthodontics, A Multimedia Textbook, pp. 38–67. Arezzo, Italy: Libra Ortodonzia.)
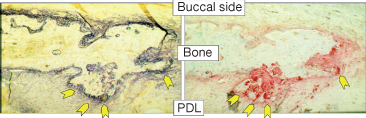
Remodelling occurs in discrete locations, involving a sequence of resorption and subsequent formation, spatially and temporally coupled, by the basic multicellular unit (BMU) (Frost 1964). Remodelling enables bone to adapt to mechanical stresses, minimizing fatigue damages, and is influenced by the action of hormones and cytokines (Frost 1998; Mori and Burr 1993). Synchronized initiation of numerous BMUs characterizes both pressure and tension sides.
The bone remodelling sequence is composed of four phases: activation, resorption, reversal and formation (Fig. 6.12). In the activation phase, lining cells change from a pancake-like to a cuboidal shape, the pre-osteoblasts, and secrete receptor activator of nuclear factor κ-B (RANK) ligand (RANKL), which remains bound to the cell surface. RANK receptors are present on cell membrane of pre-osteoclasts recruited from the bone marrow, which on activation by RANKL, fuse and differentiate into mature multinucleated osteoclasts and resorb bone. The resorption of bone also occurs as the result of inhibitory activities towards osteoblasts, as in the case of the release of sclerostin that inhibits bone formation and enhance osteoblast apoptosis (Winkler et al. 2003). At the same time, osteoprotegerin (OPG, a free-floating decoy receptor belonging to the TNF family) can bind the RANKL, thus preventing the activation of the RANK. The RANK–RANKL mechanism has been shown to be present in the PDL and is enhanced by mechanical loading (Ogasawara et al. 2004). Local delivery of RANKL has been shown to accelerate tooth movement. The up-regulation of RANKL induced by orthodontic force has been shown to be dependent on the presence of PGE2, which is released by osteoblasts and osteocytes (Kanzaki et al. 2006). Following orthodontic load, Kanzaki et al. (2004) found that the lack of OPG induced a significantly higher level of bone resorption in the compression area, while the delivery of OPG reduced tooth movement rate.
Fig. 6.12 (1) On a quiescent bone surface, osteocytes (OC) secrete sclerostin (*), which inhibits Wnt (protein that activate cell membrane receptors) signalling in cells near the surface. Pre-osteoclasts (p-OC) circulate in the bone marrow. A sudden stress results in a micro-crack. The osteocyte underneath the crack undergoes apoptosis. Osteocytes detect strains and secrete factors, including growth factors, prostaglandins and nitric oxide. LC = lining cells. (2) The stromal cells (SC) are now released from sclerostin inhibition and exposed to interleukin-1. They generate pre-osteoblasts. Stromal cells also secrete mononuclear phagocyte colony-stimulating factor (M-CSF), which helps generate the pre-osteoclasts. * = releasing factors. (3) The pre-osteoblasts proliferate and also secrete more factors, such as Wnt, interleukins and bone morphogenetic protei/>
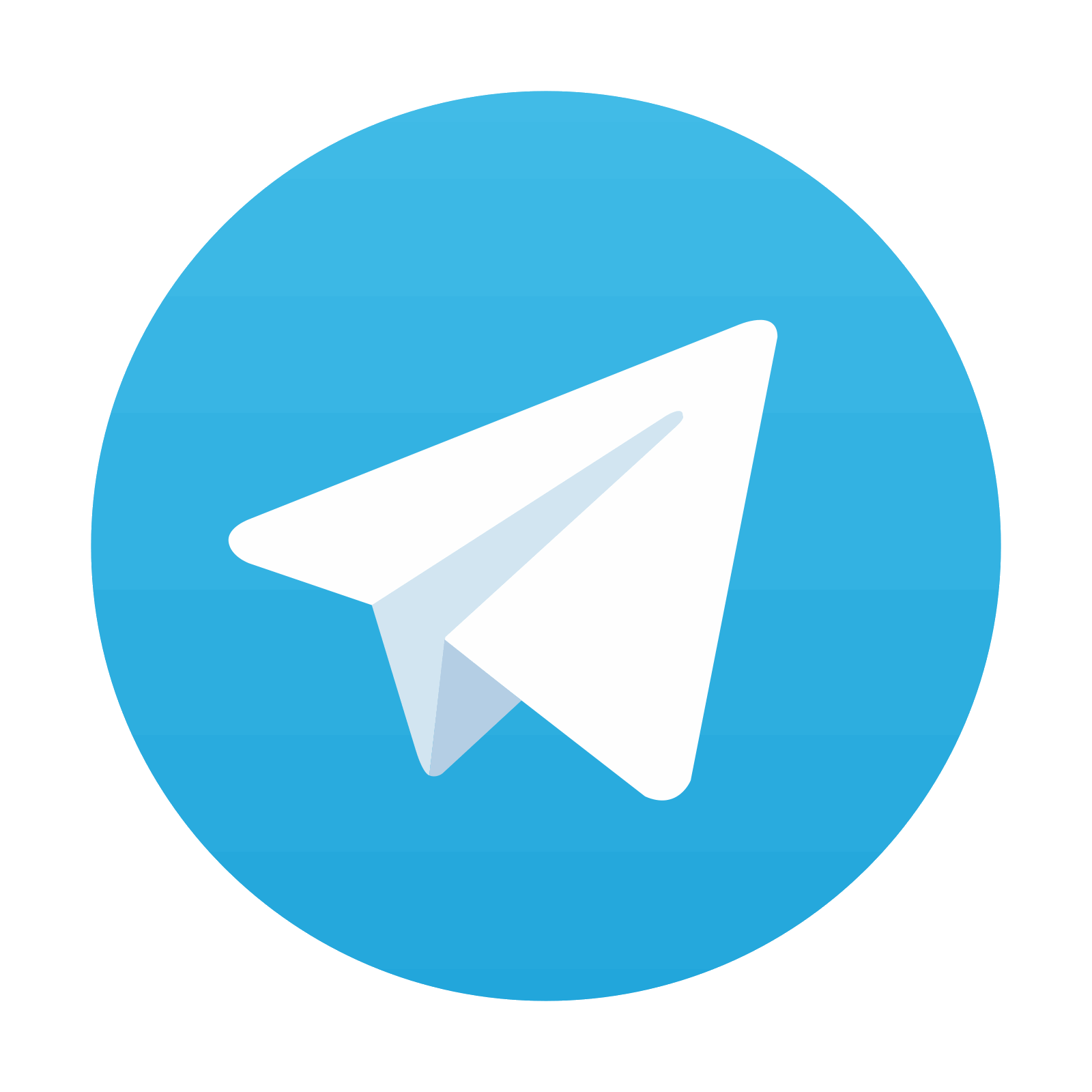
Stay updated, free dental videos. Join our Telegram channel

VIDEdental - Online dental courses
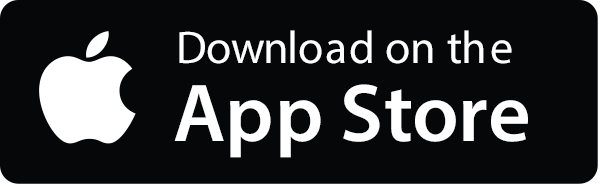
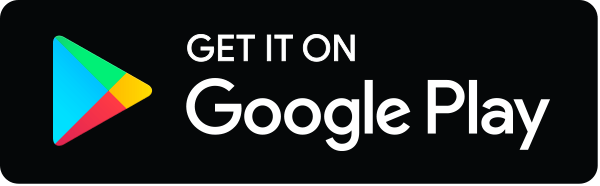