Bone Physiology, Metabolism, and Biomechanics in Implant Therapy
At the conclusion of this chapter, the reader will be able to:
. Understand the specific types of bone structure in the maxilla and mandible and the impact of these structure types on dental implant therapy.
. Understand the ways osseous physiology and the types of unique bones in the skull influence the placement, timing of loading, and role of implant therapy, depending on the anatomic site of placement.
. Understand the complex role of bone turnover (remodeling) in maintaining a functional dental implant interface.
. Understand the role of implant surface design in the healing and remodeling processes around dental implants.
The success of dental implants fundamentally depends on the anatomy, structure, physiology, and biochemistry of alveolar bone and the way it heals and then remodels around a loaded prosthesis. The outcome of implant therapy depends on osseous site development that may require hard tissue augmentation with various bone graft materials. Once this has been achieved, the treatment outcome depends on the process of osseous healing (Figure 4-1). This chapter reviews the type of dental implant–associated bone encountered in oral implant tooth replacement therapy and discusses the role of inflammation, implant biomaterials, and the complex remodeling process that maintains the implant-bone interface over the life span of the patient.
Osteology of the Maxilla and Mandible
To understand the outcomes of implant therapy, the dental practitioner must understand the bone morphology (osteology) of the craniofacial complex. A frontal section of an adult skull shows the bilateral symmetry of bone morphology and functional loading (Figures 4-2 and 4-3). As shown in Figure 4-3, the vertical components of the cranium tend to be loaded in compression (conveyed as a negative stress, by convention in engineering), and the horizontal components are loaded in tension (positive stress). This is one of the most efficient structures for achieving maximal compressive strength with minimal mass in a composite material.
Figure 4-3 Two-dimensional vector analysis of stress in the frontal section of the human skull depicted in Figure 4-2. Relative to a bilateral biting force of 100 arbitrary units, the load is distributed to the vertical components of the midface as compressive (negative) stress. The horizontal structural components are loaded in tension. In a nongrowing individual, the stress across the midpalatal suture is zero. During mastication, loads increase and the midpalatal suture is subjected to a tensile load, resulting in an increase in maxillary width. (From Atkinson SR: Balance: the magic word, Am J Orthod 50:189, 1964.)
As Figure 4-3 shows, no net tension exists across the palate in an adult. During the prenatal and early postnatal period, the palate grows in width through the posterior palatal synchondosis (primary growth center).1 This is one important reason implant placement should be delayed until skeletal maturity has been reached. Otherwise, an implant becomes positioned too far from the palatal aspect of the occlusal plane.
Although equal and opposite functional loads are delivered to the maxilla and mandible, the maxilla transfers stress to the entire cranium, whereas the mandible absorbs the entire load. Consequently, the mandible is stiffer than the maxilla. A midsagittal section through the incisors (Figure 4-4) and a frontal section through the molar region (Figure 4-5) show the distinct differences in the osseous morphology of the maxilla and mandible. This should be carefully evaluated with regard to implant placement, because the type of bone contact and proximity to the cortical plates influence when the implants can be safely loaded.
The maxilla has relatively thin cortices interconnected by a network of trabeculae (see Figures 4-2, 4-4, and 4-5). Because it is loaded primarily in compression, the maxilla is structurally similar to the body of a vertebra. The mandible, however, has thick cortices with radially oriented trabeculae (Figures 4-4 and 4-5). The structural array is similar to that for the shaft of a long bone, indicating that the mandible is loaded predominantly in bending and torsion. For this reason, mandibular flexure often influences implant prosthesis design. This biomechanical impression based on osteology is confirmed by in vivo strain gauge studies in monkeys. Hylander2,3 demonstrated substantial bending and torsion in the body of the mandible associated with normal masticatory function (Figure 4-6). This is an important issue in implant treatment planning, because implant prostheses that cross the mandibular midline or extend into the molar area undergo torsional stress. This mechanical overload can lead to prosthesis fracture, material failure, and possibly implant or implant abutment fracture.
Bone Physiology
The physiology of bone has proven elusive for investigators because of the technical limitations inherent in the study of mineralized tissues. Accurate assessment of the response of bone to mastication requires time markers (bone labels) and physiologic indexes (e.g., deoxyribonucleic acid [DNA] labels, histochemistry, and in situ hybridization) of bone cell function. Systematic investigation with these advanced methods has defined new concepts of clinically relevant bone physiology, such as mineralized sectioning, birefringence analysis, fluorescent labeling (often with tetracycline-based markers to determine rates of bone growth), microradiography, cell morphology measurements, finite elemental modeling of bone stress and strain, and electron microscopy to evaluate bone density and microarchitecture.4–19
Bone Tissue Responses to Dental Implant Placement
Woven Bone
Woven bone varies considerably in structure but is often described as a rapidly forming, cell-rich but matrix-poor tissue that forms immediately after placement of the implant. It is weak, disorganized, and poorly mineralized. However, woven bone plays a vital role in wound healing, because it (1) rapidly fills osseous defects around the implant interface, (2) provides initial continuity for fractures and osteotomy segments and also around bone grafts, and (3) strengthens bone weakened by surgery or trauma. Woven bone is the first bone formed in response to implant placement and is not found elsewhere in the adult skeleton under normal conditions. It is compacted to form composite bone, remodeled to lamellar bone, or rapidly resorbed if prematurely loaded.6,20
Lamellar Bone
Lamellar bone is a strong, highly organized, well-mineralized tissue that makes up more than 99% of the adult human skeleton. When new lamellar bone is formed, a portion of the mineral component (poorly apatite mineral) is deposited by osteoblasts during primary mineralization (Figure 4-7). Secondary mineralization, which completes the mineral component, is a physical process (based on crystal growth in the c-axis of the apatite mineral phase) that requires many months. Within physiologic limits, the strength of bone is related directly to its mineral content.21,22 The relative strengths of different histologic types of osseous tissue can be stated thusly: woven bone is weaker than new lamellar bone, which is weaker than mature lamellar bone.23 Adult human bone is almost entirely of the remodeled variety: secondary osteons and spongiosa.5,22,23 The full strength of lamellar bone to support a dental implant is not achieved until about 1 year after completion of loading (completion of the full cycle of bone resorption, woven bone formation, remodeling, and maturation of the new implant-bone interface).24,25
Composite Bone
Composite bone is an osseous tissue formed by the deposition of lamellar bone within a woven bone lattice, a process called cancellous compaction.4,26 This process is the quickest means of producing relatively strong bone.27 Composite bone is an important intermediary type of bone in the physiologic response to loading (Figure 4-8), and it is usually the predominant osseous compact bony tissue formed along the implant interface as it passes through a marrow space. When the bone is formed in the fine compaction configuration, the resulting composite of woven and lamellar bone forms structures known as primary osteons. Although composite bone may be high-quality, load-bearing osseous tissue, it is eventually remodeled into secondary osteons.5,23
Bundle Bone
Bundle bone is a functional adaptation of lamellar structure that allows the attachment of tendons and ligaments. Perpendicular striations in histologic sections, called Sharpey fibers, are the major distinguishing characteristics of bundle bone associated with dental alveolar bone. Distinct layers of bundle bone are usually seen adjacent to the periodontal ligament (PDL) (see Figure 4-8) along physiologic bone-forming surfaces.28 It is important to recall that bundle bone does not form, nor is it maintained by, dental implant placement.29
Skeletal Adaptation: Modeling and Remodeling
Skeletal adaptation to the mechanical environment is achieved through changes in (1) bone mass, (2) geometric distribution, (3) matrix organization, and (4) collagen orientation of the lamellae. In addition to these adaptive mechanisms, which influence bone formation, the mechanical properties of osseous structures change as a result of maturation, function, aging, and pathologic processes. Examples of physiologic and pathologic factors include secondary mineralization, mean bone age, fatigue damage, and loss of vitality (pathologic hypermineralization).28
Trabecular bone and cortical bone grow, adapt, and turn over by means of two fundamentally distinct mechanisms, modeling and remodeling. In bone modeling, independent sites of resorption and formation change the form of a bone (i.e., net shape or size, or both). In bone remodeling, a specific coupled sequence of resorption and formation replaces previously existing bone without a net change in size or shape. The mechanism for internal remodeling (turnover) of dense compact bone involves axially oriented cutting and filling cones composed of a coordinated set of osteoclasts and osteoblasts (Figure 4-9).4 In implant healing the initial modeling process, which is driven by inflammatory mediators, establishes the implant-bone interface.30 Later, ongoing remodeling mobilizes and deposits calcium apatite mineral by means of coupled resorption and formation: bone is resorbed and deposited at the same site.
Osteocytes, osteoblasts, osteoclasts, and possibly their precursors are thought to communicate by a complex set of growth factors.31 Transforming growth factor beta, released from bone during the resorption process, helps to stimulate subsequent bone formation to fill resorption cavities.32 Currently, growth factors released from bone are thought to mediate the coupling process through a genetic mechanism for activating and suppressing osteoclasts (RANK, RANKL, and OPG are gene products that assist in the control of remodeling). This genetic mechanism appears to be involved in the inflammatory induction of bone resorption and the coupling of bone formation at the same site (Figure 4-10).33,34
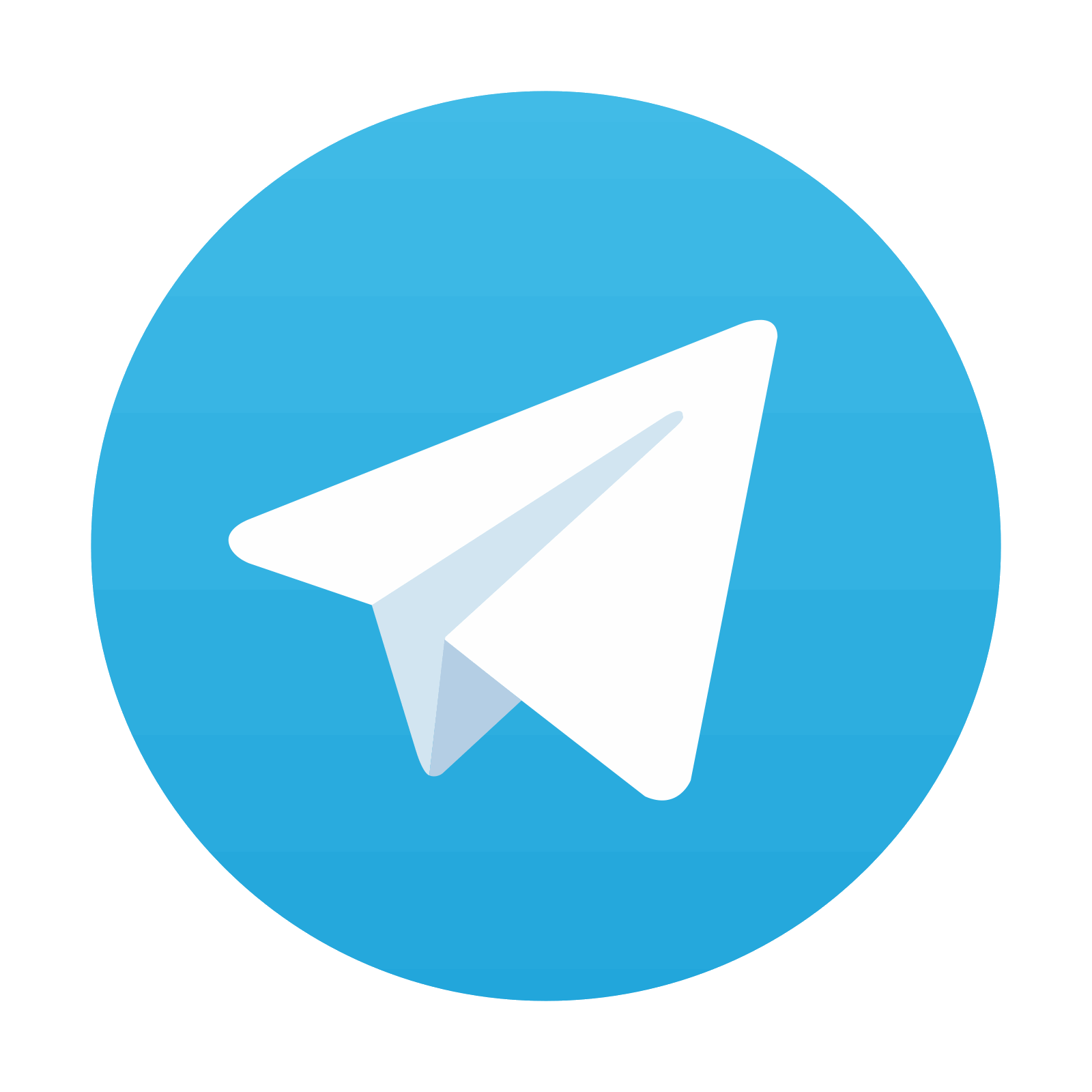
Stay updated, free dental videos. Join our Telegram channel

VIDEdental - Online dental courses
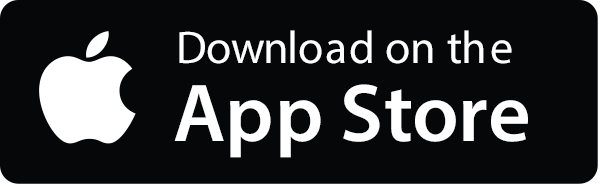
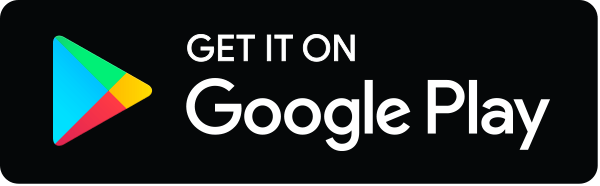