3
Cone Beam Computed Tomography in Orthodontics: Perspectives on Radiation Risk
As orthodontists and other dental specialists learn to take advantage of the benefits that three-dimensional (3D) imaging through cone beam computed tomography (CBCT) can bring for their patients, questions arise regarding the radiation risks associated with using this technology. Opinions within the profession and the public range from belief that the risk from CBCT is insignificant to great concerns about all types of radiological examinations, but particularly those used in dentistry. Claus and colleagues (2012) reported a relationship between the history of dental bitewing radiographs and brain tumors. More specifically, they noted that individuals who recalled having multiple sets of bitewings had two times greater risk of developing this type of tumor than those who had no or less frequent bitewings. This article was distributed widely via the Internet, newspapers, and magazines, resulting in many patients expressing great anxiety about the use of X-rays on themselves or their children. Although this study has been criticized due to its methodology of asking subjects about their history of dental radiographic examinations and the inherent problem of recall bias, the issues it raised ought to be of concern to every clinician who uses ionizing radiation, particularly on children.
In response to earlier criticisms in the press regarding the possible overuse of diagnostic imaging, particularly computed tomography (CT) and specifically in children, the medical profession has made a concerted effort to evaluate its approach to the utilization of imaging and put forth recommendations to guide clinicians regarding the most appropriate use of radiographic imaging. As such, the American College of Radiology has adopted the Image Wisely and Image Gently campaigns in order to obtain all the benefits of diagnostic imaging while minimizing risks. The Image Wisely program (http://www.imagewisely.org) provides guidance on selecting the most appropriate imaging examinations for specific clinical problems, while the Image Gently program (http://www.imagegently.org), developed by the Alliance for Radiation Safety in Pediatric Imaging (Goske et al., 2008) specifically focuses on imaging in children. Many professional organizations, including the American Academy of Oral and Maxillofacial Radiology, have endorsed the Image Gently campaign. Because a large proportion of an orthodontic practice involves children and adolescents, the issues of radiation dose and risk to this vulnerable population should be of great interest to orthodontists.
The purpose of this chapter is to provide the orthodontist with the necessary information to: (1) be able to select the appropriate imaging parameters to meet the goals of the diagnostic imaging examination, (2) understand the risks associated with these choices, and (3) be comfortable explaining the risks and benefits to patients and their parents. The chapter begins with a review of the basics of radiation biology and dose measurement in order to provide the necessary foundation for the discussion of these issues with regard to the use of CBCT and other imaging examinations in orthodontics. Information on doses from a variety of CBCT machines then is presented, along with a discussion of the factors that affect these doses, particularly those that the user can change. Since dose alone is not sufficient for the selection of an imaging technique, this section is followed by a short discussion on the relationship between image quality and radiation dose, and image quality and imaging goals. Finally, the chapter highlights the general radiation risk assessment for orthodontic imaging.
Radiation Effects
It did not take long after the discovery of X-rays for the first incidents of radiation injury to be reported. In those days, little information was available about how this new technology worked, either physically or biologically; early users exposed themselves, colleagues, and patients to large doses of radiation. Because X-rays are invisible, it was easy to think that nothing was happening—until tissue burns and other evidence of necrosis occurred. Early exposures were large, requiring 30 minutes or more to obtain a suitable image, and injury, at least in the form of skin reddening, was not uncommon.
As knowledge grew and the technology of image capture (film systems) and radiation shielding improved, the incidence of obvious radiation injury declined. Years after radiation was used commonly for conditions as diverse as ankylosing spondylitis, mastitis after childbirth, ringworm, tonsillitis, and acne, however, an increase in cancer incidence in tissues irradiated during these procedures was observed (National Research Council, 2006). Subsequently, research on the effects of ionizing radiation and attempts to try to minimize these effects by controlling radiation dose for diagnostic imaging increased dramatically following the aftermath of the atomic bombs dropped on Hiroshima and Nagasaki in World War II.
All forms of ionizing radiation, including X-rays, have the potential to cause cellular and tissue damage. The type and extent of damage depend on many factors, including:
- The type of radiation
- The amount or dose of radiation
- The size of the beam
- The radio-sensitivity of the tissues irradiated
- How the radiation is delivered (dose rate, fractionation)
- Sex and age of the patient
- The adequacy of the repair mechanisms of the organism.
Some consequences of radiation, such as carcinogenesis, are thought to require the interaction of radiation with other initiators and/or promoters for cancer to occur. There may be a long latent period between the time the radiation injury occurs and the time the signs or symptoms are observable. For many cancers, the latent period may be 20–30 years, making it difficult to determine the cause for any specific case of cancer. This latency also may make it easier to dismiss the idea that radiation exposure to the head during a childhood orthodontic examination might be the cause of the cancer.
Much of the basic radiation biology information currently available has been obtained through animal and tissue culture studies, while much of the information on human effects of radiation comes from case reports and epidemiologic studies of subjects who have been exposed to large doses of radiation. More recently, there have been studies looking at subjects exposed to much lower doses of radiation, such as those used in diagnostic imaging (Preston-Martin & White, 1990; Claus et al., 2012).
Much of the radiation damage to cells and tissues is a direct effect of X-ray energy on the cells, leading to ionization of molecules, along with the disruption of molecular bonds and the reformation of those bonds that often does not occur correctly. Radiation also may cause effects indirectly by ionizing other molecules in the tissue (e.g., water), leading to the production of free radicals, which then can interact with adjacent molecules to produce damage. Most of the radiation effects occur as a result of damage to the DNA that, depending on the specific injury, may lead to cell death, heritable mutations, or carcinogenesis. The body has a number of mechanisms to repair damage to DNA, but the repair is not always successful, particularly when a break in the double strand of the DNA occurs.
Radiation damage frequently is described in terms of two major types of effects: deterministic and stochastic. Deterministic effects are a result of killing of a large number of cells and typically have a threshold below which no damage is seen. Once the threshold is reached, the severity of effect is proportional to the dose. However, the probability of an effect occurring is independent of dose: once the threshold is reached, which may differ between individuals, damage will occur. Common types of deterministic effects include skin reddening, mucosal ulceration, salivary dysfunction, and cataract formation, but these typically have large thresholds and likely are seen only in large doses of radiation, such as might occur in radiation therapy or nuclear accident. It is unlikely that the doses used in diagnostic imaging, including CBCT, will cause deterministic effects.
The other major category of radiation effects, stochastic, is of concern with the use of diagnostic imaging because in this case, the probability of the effect is proportional to the dose. Stochastic effects occur as a result of sublethal damage to DNA. The cells are not killed outright, but the DNA is damaged and may not be repaired correctly. The more radiation the cells receive, the more likely nonreparable or improperly repaired damage will persist. There is no guarantee that the radiation will cause an observable effect (e.g., cancer or a heritable mutation), but the probability for these effects increases with the amount of radiation received. The severity of the effect is independent of the dose, but the probability is not. Once cancer occurs as a result of radiation, for example, the severity of the cancer is related to which cells are affected, not to the total amount of radiation. There is no threshold for stochastic effects: theoretically, a tiny amount of radiation is enough to lead to cancer, given the presence of other initiator and/or promoter factors and the lack of repair of the DNA damage.
Most biologic effects of radiation, whether deterministic or stochastic, are due to damage to the DNA, whereas a much larger dose is required to damage the non-nuclear cell cytoplasmic contents, which are more resistant to radiation. Sublethal damage to DNA can lead to mutations that vary in significance, depending on the cell type exposed. The term “genetic mutation” is used to describe alterations in the DNA occurring in reproductive cells. These mutations can be passed on to future offspring of the irradiated person, hence causing heritable mutations. Genetic mutations are not considered to be a significant risk in dental imaging because the gonads are not in the primary beam and the gonadal dose is extremely low, on the order of background radiation.
The term “somatic mutation” is used to describe DNA damage occurring in any other cells of the body (somatic cells). This damage is passed on to other cells in the same cell line, but not to offspring of the irradiated person. For example, exposure of the bone marrow can lead to the development of leukemia in the affected person, but it will not affect that person’s future children. Because leukemia is a stochastic effect, however, the exposure may not cause any problems at all; rather, it may only increase the risk of complications occurring.
Because stochastic effects involve a chance of damage occurring after irradiation, the only way to determine whether these effects occur in humans is by looking at large numbers of exposed persons, since it is unethical to expose humans deliberately or experimentally. Over the years, a large number of epidemiologic studies have been performed to evaluate the effects of radiation dose. Much of our current knowledge has been derived from the study of the people in Hiroshima and Nagasaki, Japan, who were present when the atomic bombs were dropped at the end of World War II (National Research Council, 2006). Information from radiation doses, known or calculated, and incidence of cancer in a population typically are evaluated statistically to develop a model that can then be used to predict cancer incidence from other doses, extrapolating from high doses to low doses. The choice of a statistical model then will affect the prediction of risk from radiation. It should be emphasized that this statistically derived population risk cannot be (or should not be) used to specify the risk for an individual person exposed to a certain dose. The most commonly used model is that of the linear, no threshold model, which states that the radiation risk is proportional directly to the radiation dose without a threshold. Theoretically, any dose above zero carries some risk, although the risk is small at low doses. Typically, the risk is expressed as “x” number of additional cancers beyond the normal cancer rate per million individuals per specific radiation dose, usually 1 Sievert (Sv). Some scientists feel that there are so many uncertainties in the models that the risk of cancer from low doses can be considered negligible with no cause for concern at these dose levels (Preece, 1997).
In addition to the total amount of radiation received, the way the radiation is received also may influence the amount of damage that occurs. Radiation can be delivered acutely, that is, in a relatively short period of time, such as during a dental radiographic examination, which typically requires less than 1 second for an intraoral radiograph. There can also be chronic radiation, where the dose is delivered over long periods of time; background radiation is the best example of this type of chronic radiation exposure. Humans are exposed on a continual basis to background radiation, including cosmic radiation from outer space and a variety of naturally occurring radioactive materials in the soil, building materials, food, and air. The average background radiation dose in the United States is ∼3.1 mSv/year or ∼0.008 mSv/day (8 μSv/day; National Council on Radiation Protection and Measurements [NCRP], 2009). Radiation risks from diagnostic imaging sometimes have been compared to equivalent days of background radiation, but this can be problematic due to the acute nature of diagnostic radiation exposure versus the chronic nature of background exposure. Acute exposure can overwhelm the DNA repair mechanism, leading to a higher risk of unrepaired damage than may arise from the same amount of radiation given in a low dose rate manner, as in background radiation.
The type of tissue exposed also contributes to the amount of damage occurring from a specific radiation dose. With respect to deterministic effects, the most sensitive cells are those that divide rapidly, have the longest dividing future, and are the most undifferentiated (least specialized) due to the primary effect of the radiation being on the DNA. Because of their rapidly dividing cells and long life expectancy, children are considered to have a three to five times higher risk than adults for the same radiation dose (International Commission on Radiological Protection, 1991).
With respect to radiation carcinogenesis, various tissues are more susceptible than others: colon, stomach, lung, bone marrow, and the female breast are considered highly susceptible to radiation-induced cancer; bone surface, brain, salivary glands, and skin are considered to be of low susceptibility. Other tissues are of intermediate susceptibility (White & Pharoah, 2009).
Radiation Dose Measurement
To determine radiation risks, information on radiation doses is needed. At first, that would seem to be a relatively simple task: place some type of a meter in the X-ray beam during exposure. This is a straightforward procedure for standard dental imaging, such as intraoral bitewing or lateral cephalometric radiographs. In both of these situations, there is a single exposure of the film or image receptor. However, the amount of radiation recorded on the meter does not account for the size of the X-ray beam or the type of tissue exposed, just the amount hitting the surface of the tissue. In addition, there is a slight difference between the amount of exposure and the amount actually absorbed by the tissues, although this frequently is ignored and the total amount of exposure is assumed to be absorbed.
Measuring the radiation exposure is more problematic when the X-ray beam is moving around the patient’s head over a period of several seconds, as occurs in standard dental panoramic radiography or CBCT. This is because the tissues receive variable amounts of radiation depending on their location, with no tissue receiving the entire exposure.
For these complex types of imaging examinations, radiation doses for various tissues typically are measured with a special phantom equipped with thermoluminescent dosimeters (TLDs). These devices, which are made of radiation-sensitive chemicals in the form of small chips or strips of material, absorb radiation energy and undergo a change in electron structure in the process. Later they are heated and give up the stored energy in the form of light, which can be measured and used to calculate the dose. Thus, the absorbed dose to various tissues and organs can be measured, even with a moving X-ray beam. The equivalent dose is obtained by weighting the absorbed dose by a factor related to the type of radiation used, thus allowing for mixed radiation exposure. The weighting factor for X-rays is 1. The unit for equivalent dose is Sievert (Sv), with smaller doses being designated in milliSievert (mSv, 10−3 Sv) or microSievert (μSv, 10−6 Sv).
While the equivalent dose is useful if someone wants to know the dose to a specific tissue (e.g., the brain), it can be confusing to have a long list of individual tissue/organ doses to deal with. The effective dose combines all the equivalent doses into one single term, by weighting the equivalent dose to specific tissues with a factor accounting for the radiosensitivity of the tissue and then summing the weighted doses. In a simplistic way, the effective dose can be considered almost a weighted average of the radiation dose over the entire body.
The International Commission on Radiological Protection (ICRP) has determined the weightings of the various tissues and decided which tissues should be included in the calculation of the effective dose. While the ICRP is an advisory body whose opinions do not have force of law, the scientific community nevertheless has accepted their recommendations; the effective doses for CBCT reported later in this chapter are based on the 2007 ICRP report (International Commission on Radiological Protection, 2008). Their earlier report (International Commission on Radiological Protection, 1991) was used for many years and many dose studies were published using their weighting factors. In their 2007 recommendations, which are in current use, some of the tissue weights were changed, but the biggest change affecting effective dose calculations for dental imaging arose from the reconfiguration of what is called “the remainder.” This is a collection of all tissues that do not have individual weights assigned. Also significant in the 2007 changes was the addition of the salivary glands as a weighted tissue. Since the salivary glands are in the direct X-ray beam during dental radiography, including CBCT, the effect of this change was to increase the effective dose for all dental radiographic examinations (Ludlow & Ivanovic, 2008). Thus, in comparing radiation doses from a variety of imaging techniques, such as bitewings and CBCT, it is important to know which ICRP weighting scheme was used in the calculations, 1990 or 2007, and to make sure that the data being compared use the same approaches.
Doses for Various CBCT Machines
CBCT units produce a range of sizes of field of view (FOV) from volumes as small as 4 cm × 4 cm to ones that provide complete visualization of the head and neck area (see also Chapters 1 and 2). Although there is no standard classification scheme for FOV size, it is useful to categorize fields into four ranges: large (>15 cm), medium (10–15 cm), small (8–10 cm), and dentoalveolar (4–6 cm). While some CBCT units provide a single-size FOV, others permit a variety of fields and some allow adjustable vertical collimation of the field (see Chapter 2). When other acquisition variables are held constant, reduction of the FOV results in a reduction of dose. However, there are a number of acquisition variables that, when adjusted in diffe/>
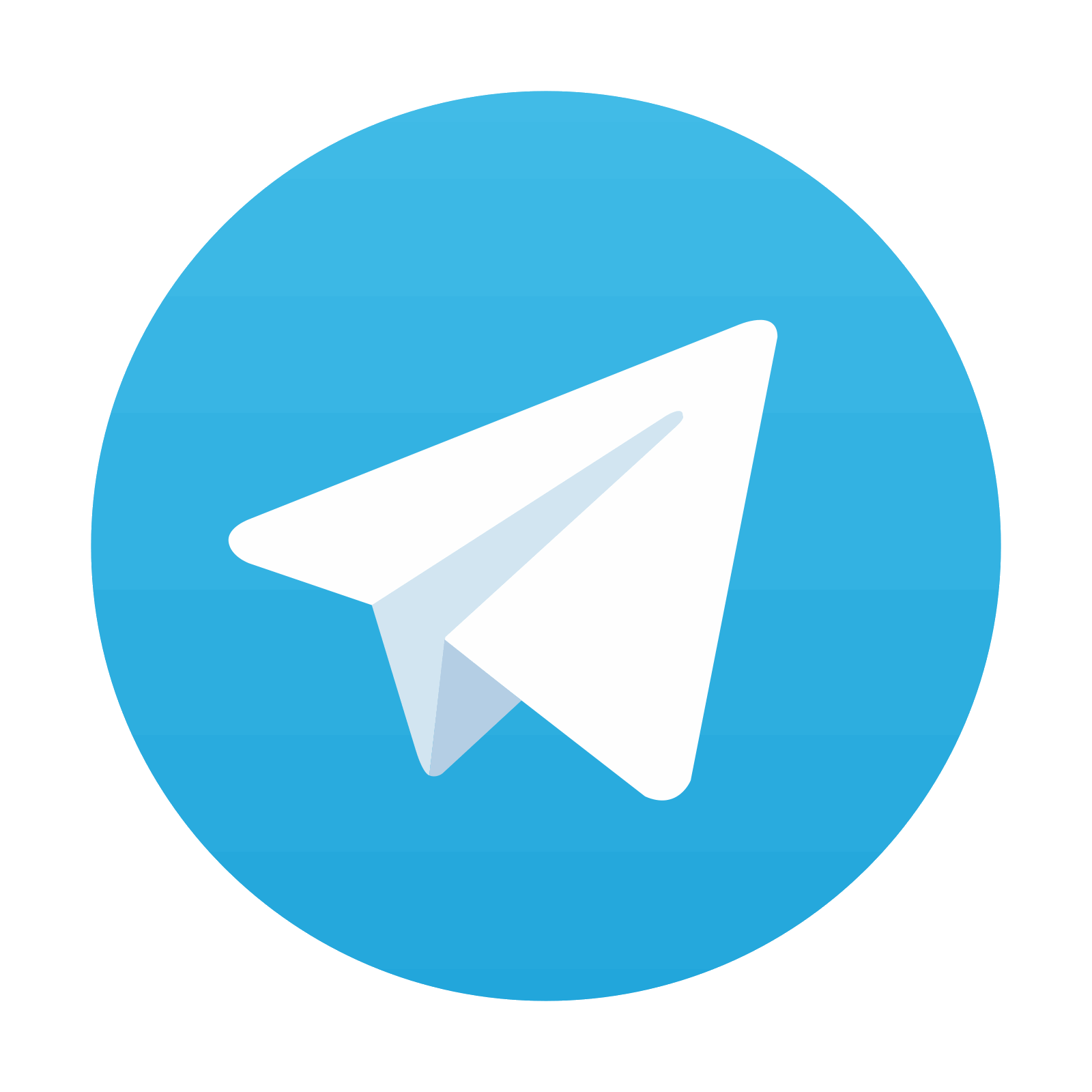
Stay updated, free dental videos. Join our Telegram channel

VIDEdental - Online dental courses
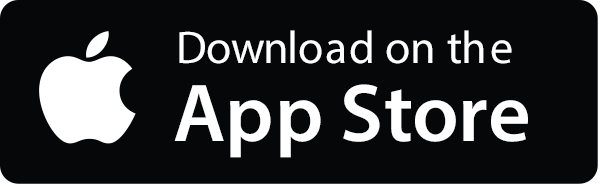
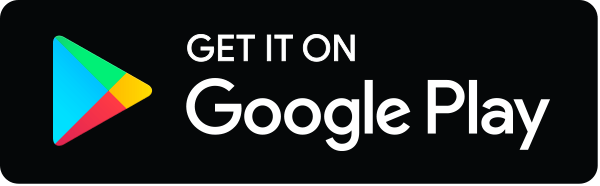