25 ALL-CERAMIC RESTORATIONS
All-ceramic inlays, onlays, veneers, and crowns can provide some of the most esthetically pleasing restorations currently available. They can be made to match natural tooth structure accurately in terms of color, surface texture, and translucency. Well-made all-ceramic restorations can be virtually indistinguishable from unrestored natural teeth (Fig. 25-1).
The importance of the design of the tooth preparation to the success of ceramic restorations cannot be overemphasized (see Chapter 11).
HISTORICAL BACKGROUND
The first attempt to use ceramics for making denture teeth was by Alexis Duchateau in 1774. More than a hundred years later, C. H. Land made the first ceramic crowns and inlays with a platinum foil matrix technique and was granted a patent in 1887.1 The popularity of ceramic restorations declined with the introduction of acrylic resin in the 1940s and continued to be low until the disadvantages of resin veneering materials (increased wear, high permeability leading to discoloration and leakage) were realized.2–4 In 1962, Weinstein and Weinstein patented a leucite-containing porcelain frit for use in metal-ceramic restorations.5 The presence of leucite, an aluminosilicate with high thermal expansion, allowed a match between the thermal expansion of the ceramic and that of the metal (see Chapter 24). The appearance of ceramic restorations was improved by the introduction of vacuum firing, which considerably reduced the amount of porosity and therefore resulted in denser and more translucent restorations than could be achieved with air firing.6
HIGH-STRENGTH CERAMICS
The chief disadvantage of the early restorations was their low strength, which limited their use to low-stress situations, such as those encountered by anterior teeth. Even so, fracture was a fairly common occurrence, which prompted the development of higher strength materials.7,8 These developments have followed two paths. One approach is to use two ceramic materials to fabricate the restoration. A high-strength but nonesthetic ceramic core material is veneered with a lower strength, esthetic porcelain. This approach is similar to the metal-ceramic technique (see Chapter 24), although the color of the ceramic core is more easily masked than that of a metal substructure. The other approach is the development of a ceramic that combines good esthetics with high strength. This has the obvious attraction of not needing the additional thickness of material to mask a high-strength core. However, at present, the strongest dental ceramics are nonesthetic core materials.
STRENGTHENING MECHANISMS OF DENTAL CERAMICS
Fabrication Defects
Fabrication defects are created during processing and consist of voids or inclusions generated during sintering. Condensation of a ceramic slurry by hand before sintering may introduce porosity. Sintering under vacuum reduces the porosity in dental ceramics from 5.6 to 0.56 volume percent.9 Porosity on the internal side of clinically failed glass-ceramic restorations has been shown to constitute a fracture initiation site.10 Also, microcracks develop within the ceramic upon cooling in leucite-containing ceramics and are caused by thermal contraction mismatch between the crystals and the glassy matrix.11–13
Surface Cracks
Surface cracks are induced by machining or grinding. The average natural flaw size varies from 20 to 50 μm.14 Usually, fracture of the ceramic material takes place from the most severe flaw, which effectively determines the fracture resistance of the restoration. Ceramic engineers analyze failure with a statistical approach, looking at flaw size and spatial distribution.15
Crystalline Reinforcement
Strengthening by crystalline reinforcement involves the introduction of a high proportion of crystalline phase into the ceramic material to improve the resistance to crack propagation. The crystals can deflect the advancing crack front to increase the fracture resistance of two-phase materials. Microstructural features that typically lead to crack deflection include (1) weakened interfaces between grains in single-phase materials that may be caused by incomplete sintering and (2) residual strains in two-phase materials.16 The latter constitutes a major issue in dental ceramics.
A crystalline phase with greater thermal expansion coefficient than the matrix produces tangential compressive stress (and radial tension) near the crystal-matrix interface. Such tangential stresses tend to divert the crack around the particle. Leucite particles have a greater thermal expansion coefficient than does the surrounding glassy matrix. Upon cooling, compressive stresses develop at the leucite crystal–matrix interface.12
Chemical Strengthening
Chemical strengthening is another method used to increase the strength of glasses and ceramics. Chemical strengthening relies on the exchange of small alkali ions for larger ions below the strain point of the ceramic material. Because stress relaxation is not possible in this temperature range, the exchange leads to the creation of a compressive layer at the surface of the ceramic.17 Finally, any applied load must first overcome this built-in compression layer before the surface can be placed into tension; this results in an increase in fracture resistance. This technique involves the use of alkali salts with a melting point lower than the glass transition temperature of the ceramic material. Ion exchange strengthening has been reported to increase the flexural strength of feldspathic dental porcelain up to 80%, depending on the ionic species involved and the composition of the porcelain.18,19 The depth of the ion-exchanged layer could be as high as 50 μm.20 However, this technique is diffusion driven, and its kinetics are limited by time, temperature, and ionic radius of the exchanged ions.
The glass industry also uses thermal tempering (fast cooling) as a strengthening method.21
Stress-Induced Transformation
In some ceramic materials such as polycrystalline zirconia, strengthening can be obtained through a stress-induced transformation. Zirconia is monoclinic at room temperature and tetragonal between about 1170° C (2140° F) and 2370° C (4300° F). The transformation between tetragonal and monoclinic zirconia is accompanied by an increase in volume. The tetragonal form can be retained at room temperature by addition of various oxides such as yttrium oxide. Stress can trigger the transformation from tetragonal to monoclinic zirconia, thereby leading to strengthening as a result of an increase in grain volume in the vicinity of the crack tip.22
Glazing
The addition of a surface glaze can also be used to strengthen ceramics. The principle is the formation of a low-expansion surface layer formed at a high temperature. Upon cooling, the low-expansion glaze places the surface of the ceramic in compression and reduces the depth and width of surface flaws.23
With contemporary dental ceramics, self-glazing is the standard technique. This consists of an additional firing in air after the original firing, without application of a low-expansion glaze. However, self-glazing does not significantly improve the flexure strength of feldspathic dental porcelain.24,25
Prevention of Stress Corrosion
The strength of ceramics is reduced in moist environments. This weakening is caused by a chemical reaction between water and the ceramic at the tip of the strength-controlling crack, resulting in an increase in the crack size—a phenomenon called stress corrosion or static fatigue.26 According to Michalske and Freiman,27 the reaction steps involve the following:
Sherrill and O’Brien28 reported a reduction in fracture strength of about 30% when dental porcelains were fractured in water, and other authors have concluded that stress corrosion is important in the performance of dental ceramic restorations.29,30
Ceramic systems such as Captek* that are baked on a metal foil may reduce fracture incidence by reducing moisture exposure to the internal surface of the ceramic material, from where the fracture is thought to initiate.10 In industry, coatings are used to reduce stress corrosion of glass and ceramics, such as optical fibers. Similar coatings have been tried experimentally for their effect on dental ceramics.31
ALL-CERAMIC SYSTEMS
The microstructure of some ceramic systems discussed in this chapter is illustrated in Figure 25-2, and their properties are summarized in Table 25-1.
Aluminous Core Ceramics
The high-strength ceramic core was first introduced to dentistry by McLean and Hughes32 in 1965. They advocated using aluminous porcelain, which is composed of aluminum oxide (alumina) crystals dispersed in a glassy matrix. Their recommendation was based on the use of alumina-reinforced porcelain in the electrical industry33 and the fact that alumina has a high fracture toughness and hardness.34
The technique devised by McLean35 involved the use of an opaque inner core containing 50% by weight alumina for high strength. This core was veneered by a combination of esthetic body and enamel porcelains with 15% and 5% crystalline alumina, respectively36 (Fig. 25-3) and matched thermal expansion. The resulting restorations were approximately 40% stronger than those with traditional feldspathic porcelain.26
Fabrication procedure
Although declining in popularity with the introduction of innovative all-ceramic products, a technician skilled in the fabrication of the aluminous core porcelain jacket crown produces an exceptionally esthetic restoration. The procedure is outlined in Figures 25-4 to 25-6.
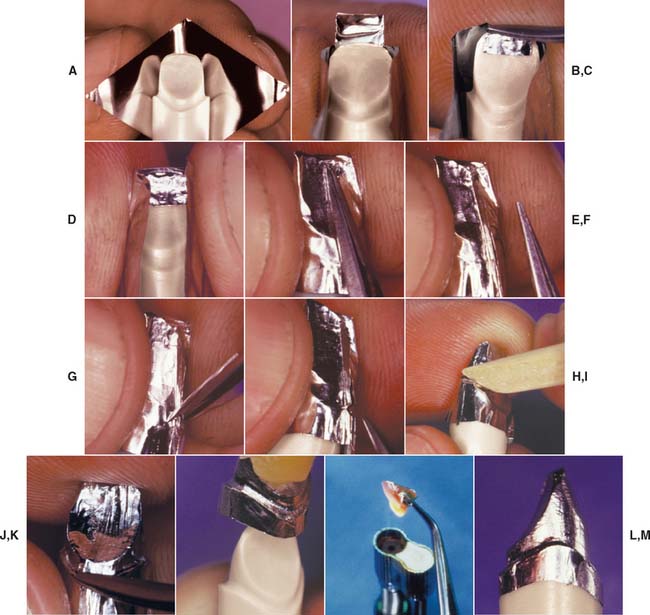
Fig. 25-4 Platinum matrix fabrication. A, A diamond-shaped foil is adapted to the facial surface (a cutting guide is provided with the foil). B, Two cuts are made, one to each incisal corner, and a triangle of foil is removed by cutting at 45 degrees toward the corners. C, The foil is folded onto the lingual surface and burnished. D and E, It is then gathered on the lingual surface with tweezers and adapted with finger pressure. F, The foil is trimmed to follow the lingual contour evenly. The two ends are separated, and one is trimmed to exactly half the width of the other. G and H, The long end is folded over the short end, and relieving cuts are made (see Fig. 25-5). Then the three-thickness joint is folded toward the short end. I, The foil is adapted with a wooden point, always starting from the incisal edge and working toward the margin. J, A beaver-tail burnisher is used to adapt the margin, working the foil toward the internal angle to prevent a perforation. Better adaptation can be achieved by swaging at this stage. The matrix is removed with sticky wax (K) and annealed in a Bunsen flame (L) to relieve work hardening. M, The completed platinum foil matrix.
Slip-Cast Ceramics
High-strength core frameworks for all-ceramic restorations can be produced with a slip-casting procedure37 such as the In-Ceram (see Fig. 25-9A).*
Slip-casting is a traditional technique in the ceramic industry and is used to make sanitary ware. The starting medium in slip-casting is a slip that is an aqueous suspension of fine ceramic particles in water with dispersing agents. The slip is applied onto a porous refractory die, which absorbs the water from the slip and leads to the condensation of the slip on the die. The piece is then fired at a high temperature (1150° C [2100°F]). The refractory die shrinks more than the condensed slip, which allows easy separation after firing. The fired porous core is later glass infiltrated, a unique process in which molten glass is drawn into the pores by capillary action at a high temperature.38 Materials processed by slip-casting tend to exhibit lower porosity and fewer processing defects than do traditionally sintered ceramic materials. The strength of In-Ceram is about three to four times greater than that of earlier alumina core materials,39,40 a finding that has prompted its use in high-stress situations such as fixed dental prostheses (FDPs) (Fig. 25-7). Two modified porcelain compositions for the In-Ceram technique have been introduced: In-Ceram Spinell* contains a magnesium spinel (MgAl2O4) as the major crystalline phase, which improves the translucency of the final restoration (Fig. 25-8). In-Ceram Zirconia contains zirconium oxide (ZrO2) and is said to provide the highest strength.41,42 Marginal fit of In-Ceram has been reported as very good43 or good44 but also poor,45 which emphasizes the technique sensitivity of the process and the need to select a skilled dental laboratory.
Fabrication procedure
Heat-Pressed Ceramics
Leucite based
Heat-pressed ceramics have been popular in restorative dentistry since the early 1990s. The restorations are waxed, invested, and pressed in a manner somewhat similar to that for gold casting. Marginal adaptation seems to be better with heat pressing than with the high-strength alumina core materials,45 although the results from individual dental laboratories may not support the research findings. Most heat-pressed materials contain leucite as a major reinforcing crystalline phase, dispersed in a glassy matrix. The crystal size varies from 3 to 10 μm, and the leucite content varies from about 35% to about 50% by volume, depending on the material. Research has shown that residual tangential stresses remain around the leucite crystals after cooling.12 Ceramic ingots are pressed at a high temperature (about 1165° C [2130° F]) into a refractory mold made by the lost-wax technique. The ceramic ingots are available in different shades. Two finishing techniques can be used: a characterization technique (surface stain only) and a layering technique, involving the application of a veneering porcelain (Fig. 25-11G and H). The two techniques lead to comparable mean flexural strength values for the resulting porcelain composite.46 The thermal expansion coefficient of the core material for the veneering technique is usually lower than that of the material for the staining technique to be compatible with the thermal expansion coefficient of the veneering porcelain. Among the currently available leucite-containing materials for heat-pressing are IPS Empress,* Optimal Pressable Ceramic,† and two lower fusing materials, Cerpress‡ and Finesse.§
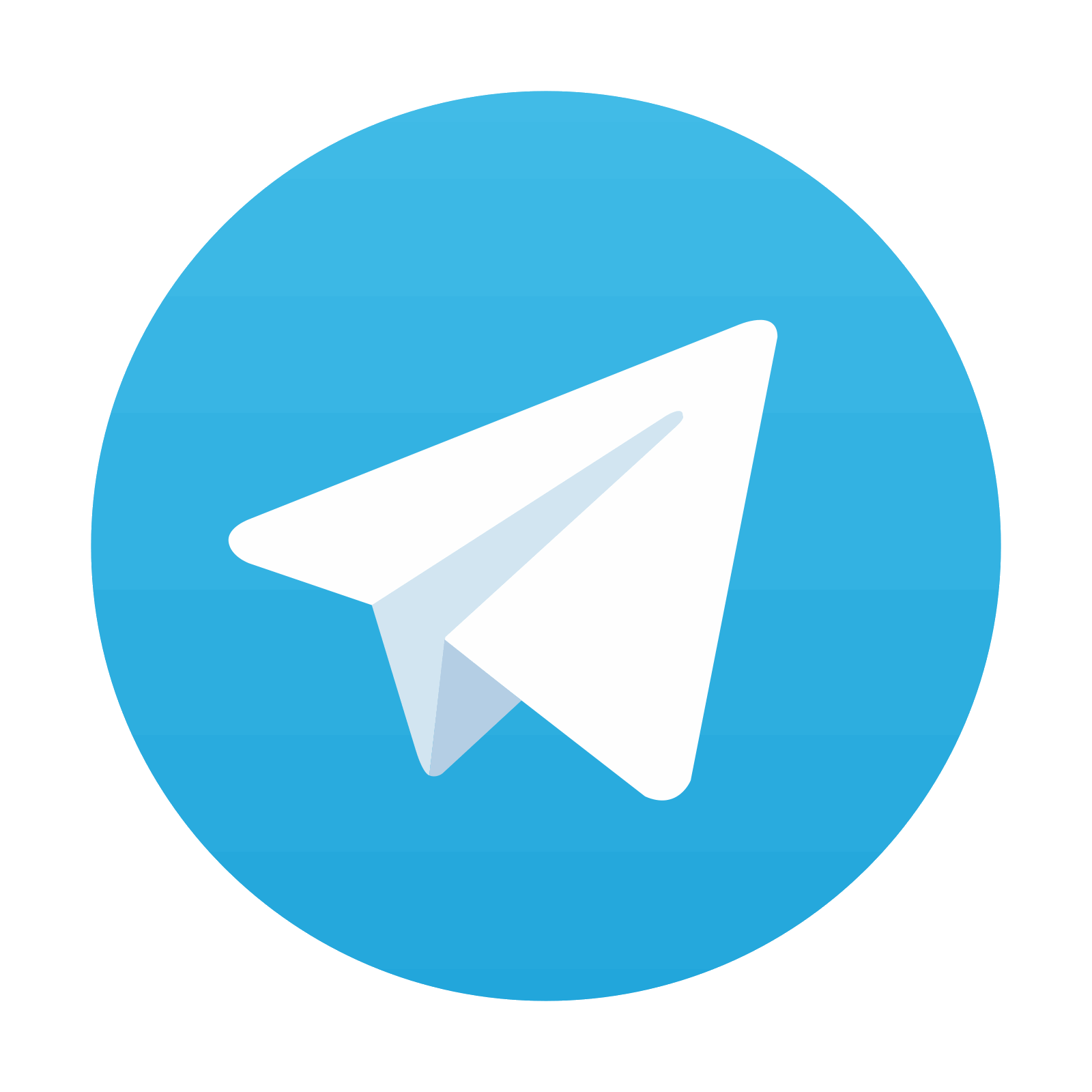
Stay updated, free dental videos. Join our Telegram channel

VIDEdental - Online dental courses
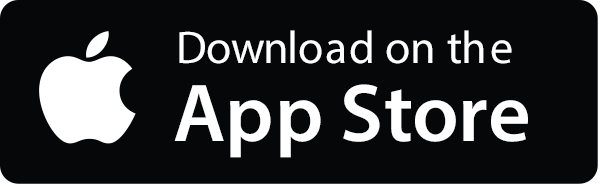
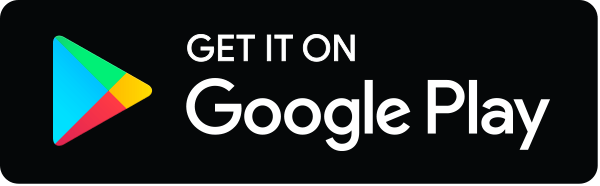